Solar energy
Solar energy is radiant light and heat from the Sun that is harnessed using a range of technologies such as solar power to generate electricity, solar thermal energy (including solar water heating), and solar architecture.[1][2]
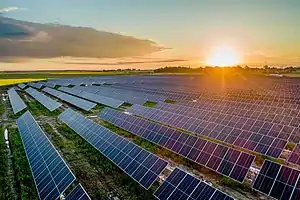
Part of a series on |
Renewable energy |
---|
![]() |
|
|
|
Part of a series on |
Sustainable energy |
---|
![]() |
|
It is an essential source of renewable energy, and its technologies are broadly characterized as either passive solar or active solar depending on how they capture and distribute solar energy or convert it into solar power. Active solar techniques include the use of photovoltaic systems, concentrated solar power, and solar water heating to harness the energy. Passive solar techniques include orienting a building to the Sun, selecting materials with favorable thermal mass or light-dispersing properties, and designing spaces that naturally circulate air.
The large magnitude of solar energy available makes it a highly appealing source of electricity. In 2020 solar energy has been the cheapest source of Electricity.[3][4] In Saudi Arabia a power purchase agreement (ppa) have been signed in April 2021 for a new solar power plant in Al-Faisaliah. The project has recorded the world’s lowest cost for Solar PV electricity production of USD 1.04 cents/ kWh.[5]
In 2011, the International Energy Agency said that "the development of affordable, inexhaustible and clean solar energy technologies will have huge longer-term benefits. It will increase countries' energy security through reliance on an indigenous, inexhaustible, and mostly import-independent resource, enhance sustainability, reduce pollution, lower the costs of mitigating global warming .... These advantages are global.".[1]
Potential
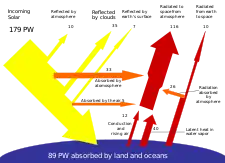
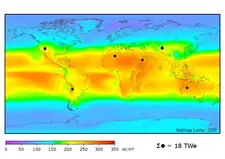
The Earth receives 174 petawatts (PW) of incoming solar radiation (insolation) at the upper atmosphere.[6] Approximately 30% is reflected back to space while the rest, 122 PW, is absorbed by clouds, oceans and land masses. The spectrum of solar light at the Earth's surface is mostly spread across the visible and near-infrared ranges with a small part in the near-ultraviolet.[7] Most of the world's population live in areas with insolation levels of 150–300 watts/m2, or 3.5–7.0 kWh/m2 per day.[8]
Solar radiation is absorbed by the Earth's land surface, oceans – which cover about 71% of the globe – and atmosphere. Warm air containing evaporated water from the oceans rises, causing atmospheric circulation or convection. When the air reaches a high altitude, where the temperature is low, water vapor condenses into clouds, which rain onto the Earth's surface, completing the water cycle. The latent heat of water condensation amplifies convection, producing atmospheric phenomena such as wind, cyclones and anticyclones.[9] Sunlight absorbed by the oceans and land masses keeps the surface at an average temperature of 14 °C.[10] By photosynthesis, green plants convert solar energy into chemically stored energy, which produces food, wood and the biomass from which fossil fuels are derived.[11]
The total solar energy absorbed by Earth's atmosphere, oceans and land masses is approximately 122 PW·year = 3,850,000 exajoules (EJ) per year.[12] In 2002 (2019), this was more energy in one hour (one hour and 25 minutes) than the world used in one year.[13][14] Photosynthesis captures approximately 3,000 EJ per year in biomass.[15]
Yearly solar fluxes & human consumption1 | ||
---|---|---|
Solar | 3,850,000 | [12] |
Wind | 2,250 | [16] |
Biomass potential | ~200 | [17] |
Primary energy use2 | 633 | [18] |
Electricity2 | ~86 | [19] |
1 Energy given in Exajoule (EJ) = 1018 J = 278 TWh 2 Consumption as of year 2019 |
The potential solar energy that could be used by humans differs from the amount of solar energy present near the surface of the planet because factors such as geography, time variation, cloud cover, and the land available to humans limit the amount of solar energy that we can acquire. In 2021, Carbon Tracker Initiative estimated the land area needed to generate all our energy from solar alone was 450,000 km2 — or about the same as the area of Sweden, or the area of Morocco, or the area of California (0.3% of the Earth's total land area).[20]
Geography affects solar energy potential because areas that are closer to the equator have a higher amount of solar radiation. However, the use of photovoltaics that can follow the position of the Sun can significantly increase the solar energy potential in areas that are farther from the equator.[21] Time variation effects the potential of solar energy because during the nighttime, there is little solar radiation on the surface of the Earth for solar panels to absorb. This limits the amount of energy that solar panels can absorb in one day. Cloud cover can affect the potential of solar panels because clouds block incoming light from the Sun and reduce the light available for solar cells.
Besides, land availability has a large effect on the available solar energy because solar panels can only be set up on land that is otherwise unused and suitable for solar panels. Roofs are a suitable place for solar cells, as many people have discovered that they can collect energy directly from their homes this way. Other areas that are suitable for solar cells are lands that are not being used for businesses where solar plants can be established.[21]
Solar technologies are characterized as either passive or active depending on the way they capture, convert and distribute sunlight and enable solar energy to be harnessed at different levels around the world, mostly depending on the distance from the equator. Although solar energy refers primarily to the use of solar radiation for practical ends, all renewable energies, other than Geothermal power and Tidal power, derive their energy either directly or indirectly from the Sun.
Active solar techniques use photovoltaics, concentrated solar power, solar thermal collectors, pumps, and fans to convert sunlight into useful outputs. Passive solar techniques include selecting materials with favorable thermal properties, designing spaces that naturally circulate air, and referencing the position of a building to the Sun. Active solar technologies increase the supply of energy and are considered supply side technologies, while passive solar technologies reduce the need for alternate resources and are generally considered demand-side technologies.[22]
In 2000, the United Nations Development Programme, UN Department of Economic and Social Affairs, and World Energy Council published an estimate of the potential solar energy that could be used by humans each year that took into account factors such as insolation, cloud cover, and the land that is usable by humans. The estimate found that solar energy has a global potential of 1,600 to 49,800 exajoules (4.4×1014 to 1.4×1016 kWh) per year (see table below).[21]
Region | North America | Latin America and Caribbean | Western Europe | Central and Eastern Europe | Former Soviet Union | Middle East and North Africa | Sub-Saharan Africa | Pacific Asia | South Asia | Centrally planned Asia | Pacific OECD |
---|---|---|---|---|---|---|---|---|---|---|---|
Minimum | 181.1 | 112.6 | 25.1 | 4.5 | 199.3 | 412.4 | 371.9 | 41.0 | 38.8 | 115.5 | 72.6 |
Maximum | 7,410 | 3,385 | 914 | 154 | 8,655 | 11,060 | 9,528 | 994 | 1,339 | 4,135 | 2,263 |
Note:
Quantitative relation of global solar potential vs. the world's primary energy consumption:
Source: United Nations Development Programme – World Energy Assessment (2000)[21] |
Thermal energy
Solar thermal technologies can be used for water heating, space heating, space cooling and process heat generation.[23]
Early commercial adaptation
In 1878, at the Universal Exposition in Paris, Augustin Mouchot successfully demonstrated a solar steam engine, but couldn't continue development because of cheap coal and other factors.
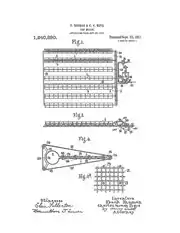
In 1897, Frank Shuman, a US inventor, engineer and solar energy pioneer built a small demonstration solar engine that worked by reflecting solar energy onto square boxes filled with ether, which has a lower boiling point than water and were fitted internally with black pipes which in turn powered a steam engine. In 1908 Shuman formed the Sun Power Company with the intent of building larger solar power plants. He, along with his technical advisor A.S.E. Ackermann and British physicist Sir Charles Vernon Boys,[24] developed an improved system using mirrors to reflect solar energy upon collector boxes, increasing heating capacity to the extent that water could now be used instead of ether. Shuman then constructed a full-scale steam engine powered by low-pressure water, enabling him to patent the entire solar engine system by 1912.
Shuman built the world's first solar thermal power station in Maadi, Egypt, between 1912 and 1913. His plant used parabolic troughs to power a 45–52 kilowatts (60–70 hp) engine that pumped more than 22,000 litres (4,800 imp gal; 5,800 US gal) of water per minute from the Nile River to adjacent cotton fields. Although the outbreak of World War I and the discovery of cheap oil in the 1930s discouraged the advancement of solar energy, Shuman's vision, and basic design were resurrected in the 1970s with a new wave of interest in solar thermal energy.[25] In 1916 Shuman was quoted in the media advocating solar energy's utilization, saying:
We have proved the commercial profit of sun power in the tropics and have more particularly proved that after our stores of oil and coal are exhausted the human race can receive unlimited power from the rays of the Sun.
— Frank Shuman, New York Times, 2 July 1916[26]
Water heating
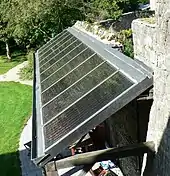
Solar hot water systems use sunlight to heat water. In middle geographical latitudes (between 40 degrees north and 40 degrees south), 60 to 70% of the domestic hot water use, with water temperatures up to 60 °C (140 °F), can be provided by solar heating systems.[27] The most common types of solar water heaters are evacuated tube collectors (44%) and glazed flat plate collectors (34%) generally used for domestic hot water; and unglazed plastic collectors (21%) used mainly to heat swimming pools.[28]
As of 2015, the total installed capacity of solar hot water systems was approximately 436 thermal gigawatt (GWth), and China is the world leader in their deployment with 309 GWth installed, taken up 71% of the market.[29] Israel and Cyprus are the per capita leaders in the use of solar hot water systems with over 90% of homes using them.[30] In the United States, Canada, and Australia, heating swimming pools is the dominant application of solar hot water with an installed capacity of 18 GWth as of 2005.[22]
Heating, cooling and ventilation
In the United States, heating, ventilation and air conditioning (HVAC) systems account for 30% (4.65 EJ/yr) of the energy used in commercial buildings and nearly 50% (10.1 EJ/yr) of the energy used in residential buildings.[31][32] Solar heating, cooling and ventilation technologies can be used to offset a portion of this energy. Use of solar for heating can roughly be divided into passive solar concepts and active solar concepts, depending on whether active elements such as sun tracking and solar concentrator optics are used.
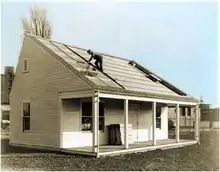
Thermal mass is any material that can be used to store heat—heat from the Sun in the case of solar energy. Common thermal mass materials include stone, cement, and water. Historically they have been used in arid climates or warm temperate regions to keep buildings cool by absorbing solar energy during the day and radiating stored heat to the cooler atmosphere at night. However, they can be used in cold temperate areas to maintain warmth as well. The size and placement of thermal mass depend on several factors such as climate, daylighting, and shading conditions. When duly incorporated, thermal mass maintains space temperatures in a comfortable range and reduces the need for auxiliary heating and cooling equipment.[33]
A solar chimney (or thermal chimney, in this context) is a passive solar ventilation system composed of a vertical shaft connecting the interior and exterior of a building. As the chimney warms, the air inside is heated, causing an updraft that pulls air through the building. Performance can be improved by using glazing and thermal mass materials[34] in a way that mimics greenhouses.
Deciduous trees and plants have been promoted as a means of controlling solar heating and cooling. When planted on the southern side of a building in the northern hemisphere or the northern side in the southern hemisphere, their leaves provide shade during the summer, while the bare limbs allow light to pass during the winter.[35] Since bare, leafless trees shade 1/3 to 1/2 of incident solar radiation, there is a balance between the benefits of summer shading and the corresponding loss of winter heating.[36] In climates with significant heating loads, deciduous trees should not be planted on the Equator-facing side of a building because they will interfere with winter solar availability. They can, however, be used on the east and west sides to provide a degree of summer shading without appreciably affecting winter solar gain.[37]
Cooking
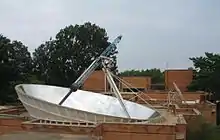
Solar cookers use sunlight for cooking, drying, and pasteurization. They can be grouped into three broad categories: box cookers, panel cookers, and reflector cookers.[38] The simplest solar cooker is the box cooker first built by Horace de Saussure in 1767.[39] A basic box cooker consists of an insulated container with a transparent lid. It can be used effectively with partially overcast skies and will typically reach temperatures of 90–150 °C (194–302 °F).[40] Panel cookers use a reflective panel to direct sunlight onto an insulated container and reach temperatures comparable to box cookers. Reflector cookers use various concentrating geometries (dish, trough, Fresnel mirrors) to focus light on a cooking container. These cookers reach temperatures of 315 °C (599 °F) and above but require direct light to function properly and must be repositioned to track the Sun.[41]
Process heat
Solar concentrating technologies such as parabolic dish, trough and Scheffler reflectors can provide process heat for commercial and industrial applications. The first commercial system was the Solar Total Energy Project (STEP) in Shenandoah, Georgia, US where a field of 114 parabolic dishes provided 50% of the process heating, air conditioning and electrical requirements for a clothing factory. This grid-connected cogeneration system provided 400 kW of electricity plus thermal energy in the form of 401 kW steam and 468 kW chilled water, and had a one-hour peak load thermal storage.[42] Evaporation ponds are shallow pools that concentrate dissolved solids through evaporation. The use of evaporation ponds to obtain salt from seawater is one of the oldest applications of solar energy. Modern uses include concentrating brine solutions used in leach mining and removing dissolved solids from waste streams.[43]
Clothes lines, clotheshorses, and clothes racks dry clothes through evaporation by wind and sunlight without consuming electricity or gas. In some states of the United States legislation protects the "right to dry" clothes.[44] Unglazed transpired collectors (UTC) are perforated sun-facing walls used for preheating ventilation air. UTCs can raise the incoming air temperature up to 22 °C (40 °F) and deliver outlet temperatures of 45–60 °C (113–140 °F).[45] The short payback period of transpired collectors (3 to 12 years) makes them a more cost-effective alternative than glazed collection systems.[45] As of 2003, over 80 systems with a combined collector area of 35,000 square metres (380,000 sq ft) had been installed worldwide, including an 860 m2 (9,300 sq ft) collector in Costa Rica used for drying coffee beans and a 1,300 m2 (14,000 sq ft) collector in Coimbatore, India, used for drying marigolds.[46]
Water treatment
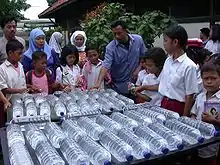
Solar distillation can be used to make saline or brackish water potable. The first recorded instance of this was by 16th-century Arab alchemists.[47] A large-scale solar distillation project was first constructed in 1872 in the Chilean mining town of Las Salinas.[48] The plant, which had solar collection area of 4,700 m2 (51,000 sq ft), could produce up to 22,700 L (5,000 imp gal; 6,000 US gal) per day and operate for 40 years.[48] Individual still designs include single-slope, double-slope (or greenhouse type), vertical, conical, inverted absorber, multi-wick, and multiple effect. These stills can operate in passive, active, or hybrid modes. Double-slope stills are the most economical for decentralized domestic purposes, while active multiple effect units are more suitable for large-scale applications.[47]
Solar water disinfection (SODIS) involves exposing water-filled plastic polyethylene terephthalate (PET) bottles to sunlight for several hours.[49] Exposure times vary depending on weather and climate from a minimum of six hours to two days during fully overcast conditions.[50] It is recommended by the World Health Organization as a viable method for household water treatment and safe storage.[51] Over two million people in developing countries use this method for their daily drinking water.[50]
Solar energy may be used in a water stabilization pond to treat waste water without chemicals or electricity. A further environmental advantage is that algae grow in such ponds and consume carbon dioxide in photosynthesis, although algae may produce toxic chemicals that make the water unusable.[52][53]
Molten salt technology
Molten salt can be employed as a thermal energy storage method to retain thermal energy collected by a solar tower or solar trough of a concentrated solar power plant so that it can be used to generate electricity in bad weather or at night. It was demonstrated in the Solar Two project from 1995 to 1999. The system is predicted to have an annual efficiency of 99%, a reference to the energy retained by storing heat before turning it into electricity, versus converting heat directly into electricity.[54][55][56] The molten salt mixtures vary. The most extended mixture contains sodium nitrate, potassium nitrate and calcium nitrate. It is non-flammable and non-toxic, and has already been used in the chemical and metals industries as a heat-transport fluid. Hence, experience with such systems exists in non-solar applications.
The salt melts at 131 °C (268 °F). It is kept liquid at 288 °C (550 °F) in an insulated "cold" storage tank. The liquid salt is pumped through panels in a solar collector where the focused irradiance heats it to 566 °C (1,051 °F). It is then sent to a hot storage tank. This is so well insulated that the thermal energy can be usefully stored for up to a week.[57]
When electricity is needed, the hot salt is pumped to a conventional steam-generator to produce superheated steam for a turbine/generator as used in any conventional coal, oil, or nuclear power plant. A 100-megawatt turbine would need a tank about 9.1 metres (30 ft) tall and 24 metres (79 ft) in diameter to drive it for four hours by this design.
Several parabolic trough power plants in Spain[58] and solar power tower developer SolarReserve use this thermal energy storage concept. The Solana Generating Station in the U.S. has six hours of storage by molten salt. The María Elena plant[59] is a 400 MW thermo-solar complex in the northern Chilean region of Antofagasta employing molten salt technology.
Electricity production
Solar power is the conversion of energy from sunlight into electricity, either directly using photovoltaics (PV), indirectly using concentrated solar power, or a combination. Photovoltaic cells convert light into an electric current using the photovoltaic effect.[60] Concentrated solar power systems use lenses or mirrors and solar tracking systems to focus a large area of sunlight to a hot spot, often to drive a steam turbine.
Photovoltaics were initially solely used as a source of electricity for small and medium-sized applications, from the calculator powered by a single solar cell to remote homes powered by an off-grid rooftop PV system. Commercial concentrated solar power plants were first developed in the 1980s. Since then, as the cost of solar electricity has fallen, grid-connected solar PV systems have grown more or less exponentially. Millions of installations and gigawatt-scale photovoltaic power stations continue to be built. Solar PV has rapidly become a viable low-carbon technology, and since 2020, provides the cheapest source of electricity in history.[61]
As of 2021, solar generates 4% of the world's electricity, compared to 1% in 2015 when the Paris Agreement to limit climate change was signed.[62] Along with onshore wind, the cheapest levelised cost of electricity is utility-scale solar.[63] The International Energy Agency said in 2021 that under its "Net Zero by 2050" scenario solar power would contribute about 20% of worldwide energy consumption, and solar would be the world's largest source of electricity.[64]Photovoltaics
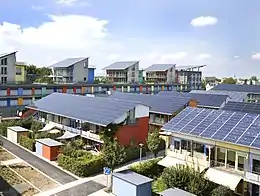
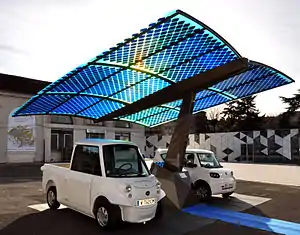
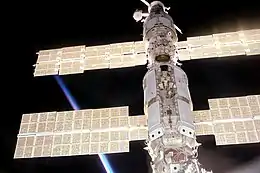
A photovoltaic system employs solar modules, each comprising a number of solar cells, which generate electrical power. PV installations may be ground-mounted, rooftop-mounted, wall-mounted or floating. The mount may be fixed or use a solar tracker to follow the sun across the sky.
Photovoltaic technology helps to mitigate climate change because it emits much less carbon dioxide than fossil fuels. Solar PV has specific advantages as an energy source: once installed, its operation generates no pollution and no greenhouse gas emissions, it shows scalability in respect of power needs and silicon has large availability in the Earth's crust, although other materials required in PV system manufacture such as silver may constrain further growth in the technology. Other major constraints identified are competition for land use.[65] The use of PV as a main source requires energy storage systems or global distribution by high-voltage direct current power lines causing additional costs, and also has a number of other specific disadvantages such as variable power generation which have to be balanced. Production and installation does cause some pollution and greenhouse gas emissions, though only a fraction of the emissions caused by fossil fuels.
Photovoltaic systems have long been used in specialized applications as stand-alone installations and grid-connected PV systems have been in use since the 1990s.[66] Photovoltaic modules were first mass-produced in 2000, when the German government funded a one hundred thousand roof program.[67] Decreasing costs has allowed PV to grow as an energy source. This has been partially driven by massive Chinese government investment in developing solar production capacity since 2000, and achieving economies of scale. Improvements in manufacturing technology and efficiency have also led to decreasing costs.[68][69] Net metering and financial incentives, such as preferential feed-in tariffs for solar-generated electricity, have supported solar PV installations in many countries.[70] Panel prices dropped by a factor of 4 between 2004 and 2011. Module prices dropped 90% of over the 2010s.
In 2019, worldwide installed PV capacity increased to more than 635 gigawatts (GW) covering approximately two percent of global electricity demand.[71] After hydro and wind powers, PV is the third renewable energy source in terms of global capacity. In 2019 the International Energy Agency expected a growth by 700–880 GW from 2019 to 2024.[72] In some instances, PV has offered the cheapest source of electrical power in regions with a high solar potential, with a bid for pricing as low as 0.01567 US$/kWh in Qatar in 2020.[73] In 2020 the International Energy Agency stated in its World Energy Outlook that ‘[f]or projects with low cost financing that tap high quality resources, solar PV is now the cheapest source of electricity in history.[74]Concentrated solar power
Concentrating Solar Power (CSP) systems use lenses or mirrors and tracking systems to focus a large area of sunlight into a small beam. The concentrated heat is then used as a heat source for a conventional power plant. A wide range of concentrating technologies exists; the most developed are the parabolic trough, the solar tower collectors, the concentrating linear Fresnel reflector, and the Stirling dish. Various techniques are used to track the Sun and focus light. In all of these systems, a working fluid is heated by the concentrated sunlight, and is then used for power generation or energy storage.[75] Designs need to account for the risk of a dust storm, hail, or another extreme weather event that can damage the fine glass surfaces of solar power plants. Metal grills would allow a high percentage of sunlight to enter the mirrors and solar panels while also preventing most damage.
Architecture and urban planning
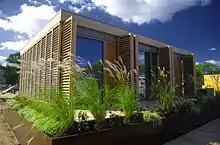
Sunlight has influenced building design since the beginning of architectural history.[77] Advanced solar architecture and urban planning methods were first employed by the Greeks and Chinese, who oriented their buildings toward the south to provide light and warmth.[78]
The common features of passive solar architecture are orientation relative to the Sun, compact proportion (a low surface area to volume ratio), selective shading (overhangs) and thermal mass.[77] When these features are tailored to the local climate and environment, they can produce well-lit spaces that stay in a comfortable temperature range. Socrates' Megaron House is a classic example of passive solar design.[77] The most recent approaches to solar design use computer modeling tying together solar lighting, heating and ventilation systems in an integrated solar design package.[79] Active solar equipment such as pumps, fans, and switchable windows can complement passive design and improve system performance.
Urban heat islands (UHI) are metropolitan areas with higher temperatures than that of the surrounding environment. The higher temperatures result from increased absorption of solar energy by urban materials such as asphalt and concrete, which have lower albedos and higher heat capacities than those in the natural environment. A straightforward method of counteracting the UHI effect is to paint buildings and roads white and to plant trees in the area. Using these methods, a hypothetical "cool communities" program in Los Angeles has projected that urban temperatures could be reduced by approximately 3 °C at an estimated cost of US$1 billion, giving estimated total annual benefits of US$530 million from reduced air-conditioning costs and healthcare savings.[80]
Agriculture and horticulture
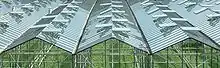
Agriculture and horticulture seek to optimize the capture of solar energy to optimize the productivity of plants. Techniques such as timed planting cycles, tailored row orientation, staggered heights between rows and the mixing of plant varieties can improve crop yields.[81][82] While sunlight is generally considered a plentiful resource, the exceptions highlight the importance of solar energy to agriculture. During the short growing seasons of the Little Ice Age, French and English farmers employed fruit walls to maximize the collection of solar energy. These walls acted as thermal masses and accelerated ripening by keeping plants warm. Early fruit walls were built perpendicular to the ground and facing south, but over time, sloping walls were developed to make better use of sunlight. In 1699, Nicolas Fatio de Duillier even suggested using a tracking mechanism which could pivot to follow the Sun.[83] Applications of solar energy in agriculture aside from growing crops include pumping water, drying crops, brooding chicks and drying chicken manure.[46][84] More recently the technology has been embraced by vintners, who use the energy generated by solar panels to power grape presses.[85]
Greenhouses convert solar light to heat, enabling year-round production and the growth (in enclosed environments) of specialty crops and other plants not naturally suited to the local climate. Primitive greenhouses were first used during Roman times to produce cucumbers year-round for the Roman emperor Tiberius.[86] The first modern greenhouses were built in Europe in the 16th century to keep exotic plants brought back from explorations abroad.[87] Greenhouses remain an important part of horticulture today. Plastic transparent materials have also been used to similar effect in polytunnels and row covers.
Transport
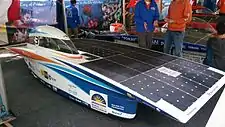
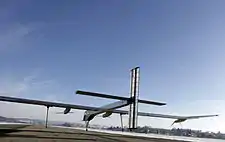
Development of a solar-powered car has been an engineering goal since the 1980s. The World Solar Challenge is a biannual solar-powered car race, where teams from universities and enterprises compete over 3,021 kilometres (1,877 mi) across central Australia from Darwin to Adelaide. In 1987, when it was founded, the winner's average speed was 67 kilometres per hour (42 mph) and by 2007 the winner's average speed had improved to 90.87 kilometres per hour (56.46 mph).[88] The North American Solar Challenge and the planned South African Solar Challenge are comparable competitions that reflect an international interest in the engineering and development of solar powered vehicles.[89][90]
Some vehicles use solar panels for auxiliary power, such as for air conditioning, to keep the interior cool, thus reducing fuel consumption.[91][92]
In 1975, the first practical solar boat was constructed in England.[93] By 1995, passenger boats incorporating PV panels began appearing and are now used extensively.[94] In 1996, Kenichi Horie made the first solar-powered crossing of the Pacific Ocean, and the Sun21 catamaran made the first solar-powered crossing of the Atlantic Ocean in the winter of 2006–2007.[95] There were plans to circumnavigate the globe in 2010.[96]
In 1974, the unmanned AstroFlight Sunrise airplane made the first solar flight. On 29 April 1979, the Solar Riser made the first flight in a solar-powered, fully controlled, man-carrying flying machine, reaching an altitude of 40 ft (12 m). In 1980, the Gossamer Penguin made the first piloted flights powered solely by photovoltaics. This was quickly followed by the Solar Challenger which crossed the English Channel in July 1981. In 1990 Eric Scott Raymond in 21 hops flew from California to North Carolina using solar power.[97] Developments then turned back to unmanned aerial vehicles (UAV) with the Pathfinder (1997) and subsequent designs, culminating in the Helios which set the altitude record for a non-rocket-propelled aircraft at 29,524 metres (96,864 ft) in 2001.[98] The Zephyr, developed by BAE Systems, is the latest in a line of record-breaking solar aircraft, making a 54-hour flight in 2007, and month-long flights were envisioned by 2010.[99] As of 2016, Solar Impulse, an electric aircraft, is currently circumnavigating the globe. It is a single-seat plane powered by solar cells and capable of taking off under its own power. The design allows the aircraft to remain airborne for several days.[100]
A solar balloon is a black balloon that is filled with ordinary air. As sunlight shines on the balloon, the air inside is heated and expands, causing an upward buoyancy force, much like an artificially heated hot air balloon. Some solar balloons are large enough for human flight, but usage is generally limited to the toy market as the surface-area to payload-weight ratio is relatively high.[101]
Fuel production
.jpg.webp)
Solar chemical processes use solar energy to drive chemical reactions. These processes offset energy that would otherwise come from a fossil fuel source and can also convert solar energy into storable and transportable fuels. Solar induced chemical reactions can be divided into thermochemical or photochemical.[102] A variety of fuels can be produced by artificial photosynthesis.[103] The multielectron catalytic chemistry involved in making carbon-based fuels (such as methanol) from reduction of carbon dioxide is challenging; a feasible alternative is hydrogen production from protons, though use of water as the source of electrons (as plants do) requires mastering the multielectron oxidation of two water molecules to molecular oxygen.[104] Some have envisaged working solar fuel plants in coastal metropolitan areas by 2050 – the splitting of seawater providing hydrogen to be run through adjacent fuel-cell electric power plants and the pure water by-product going directly into the municipal water system.[105]
Hydrogen production technologies have been a significant area of solar chemical research since the 1970s. Aside from electrolysis driven by photovoltaic or photochemical cells, several thermochemical processes have also been explored. One such route uses concentrators to split water into oxygen and hydrogen at high temperatures (2,300–2,600 °C or 4,200–4,700 °F).[106] Another approach uses the heat from solar concentrators to drive the steam reformation of natural gas thereby increasing the overall hydrogen yield compared to conventional reforming methods.[107] Thermochemical cycles characterized by the decomposition and regeneration of reactants present another avenue for hydrogen production. The Solzinc process under development at the Weizmann Institute of Science uses a 1 MW solar furnace to decompose zinc oxide (ZnO) at temperatures above 1,200 °C (2,200 °F). This initial reaction produces pure zinc, which can subsequently be reacted with water to produce hydrogen.[108]
Energy storage methods
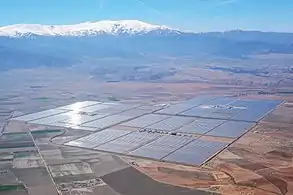
Thermal mass systems can store solar energy in the form of heat at domestically useful temperatures for daily or interseasonal durations. Thermal storage systems generally use readily available materials with high specific heat capacities such as water, earth and stone. Well-designed systems can lower peak demand, shift time-of-use to off-peak hours and reduce overall heating and cooling requirements.[109][110]
Phase change materials such as paraffin wax and Glauber's salt are another thermal storage medium. These materials are inexpensive, readily available, and can deliver domestically useful temperatures (approximately 64 °C or 147 °F). The "Dover House" (in Dover, Massachusetts) was the first to use a Glauber's salt heating system, in 1948.[111] Solar energy can also be stored at high temperatures using molten salts. Salts are an effective storage medium because they are low-cost, have a high specific heat capacity, and can deliver heat at temperatures compatible with conventional power systems. The Solar Two project used this method of energy storage, allowing it to store 1.44 terajoules (400,000 kWh) in its 68 m³ storage tank with an annual storage efficiency of about 99%.[112]
Off-grid PV systems have traditionally used rechargeable batteries to store excess electricity. With grid-tied systems, excess electricity can be sent to the transmission grid, while standard grid electricity can be used to meet shortfalls. Net metering programs give household systems credit for any electricity they deliver to the grid. This is handled by 'rolling back' the meter whenever the home produces more electricity than it consumes. If the net electricity use is below zero, the utility then rolls over the kilowatt-hour credit to the next month.[113] Other approaches involve the use of two meters, to measure electricity consumed vs. electricity produced. This is less common due to the increased installation cost of the second meter. Most standard meters accurately measure in both directions, making a second meter unnecessary.
Pumped-storage hydroelectricity stores energy in the form of water pumped when energy is available from a lower elevation reservoir to a higher elevation one. The energy is recovered when demand is high by releasing the water, with the pump becoming a hydroelectric power generator.[114]
Development, deployment and economics
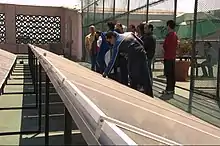
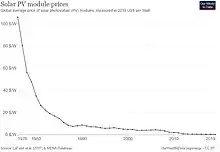
Beginning with the surge in coal use, which accompanied the Industrial Revolution, energy consumption has steadily transitioned from wood and biomass to fossil fuels. The early development of solar technologies starting in the 1860s was driven by an expectation that coal would soon become scarce. However, development of solar technologies stagnated in the early 20th century in the face of the increasing availability, economy, and utility of coal and petroleum.[115]
The 1973 oil embargo and 1979 energy crisis caused a reorganization of energy policies around the world. It brought renewed attention to developing solar technologies.[116][117] Deployment strategies focused on incentive programs such as the Federal Photovoltaic Utilization Program in the US and the Sunshine Program in Japan. Other efforts included the formation of research facilities in the US (SERI, now NREL), Japan (NEDO), and Germany (Fraunhofer Institute for Solar Energy Systems ISE).[118]
Commercial solar water heaters began appearing in the United States in the 1890s.[119] These systems saw increasing use until the 1920s but were gradually replaced by cheaper and more reliable heating fuels.[120] As with photovoltaics, solar water heating attracted renewed attention as a result of the oil crises in the 1970s, but interest subsided in the 1980s due to falling petroleum prices. Development in the solar water heating sector progressed steadily throughout the 1990s, and annual growth rates have averaged 20% since 1999.[121] Although generally underestimated, solar water heating and cooling is by far the most widely deployed solar technology with an estimated capacity of 154 GW as of 2007.[121]
The International Energy Agency has said that solar energy can make considerable contributions to solving some of the most urgent problems the world now faces:[1]
The development of affordable, inexhaustible, and clean solar energy technologies will have huge longer-term benefits. It will increase countries' energy security through reliance on an indigenous, inexhaustible, and mostly import-independent resource, enhance sustainability, reduce pollution, lower the costs of mitigating climate change, and keep fossil fuel prices lower than otherwise. These advantages are global. Hence the additional costs of the incentives for early deployment should be considered learning investments; they must be wisely spent and need to be widely shared.[1]
In 2011, a report by the International Energy Agency found that solar energy technologies such as photovoltaics, solar hot water, and concentrated solar power could provide a third of the world's energy by 2060 if politicians commit to limiting climate change and transitioning to renewable energy. The energy from the Sun could play a key role in de-carbonizing the global economy alongside improvements in energy efficiency and imposing costs on greenhouse gas emitters. "The strength of solar is the incredible variety and flexibility of applications, from small scale to big scale".[122]
We have proved ... that after our stores of oil and coal are exhausted the human race can receive unlimited power from the rays of the Sun.
— Frank Shuman, The New York Times, 2 July 1916.[26]
In 2021 Lazard estimated the levelized cost of new build unsubsidized utility scale solar electricity at less than 37 dollars per MWh and existing coal-fired power above that amount.[3][4] The 2021 report also said that new solar was also cheaper than new gas-fired power, but not generally existing gas power.[4]
Experimental solar power
Concentrated photovoltaics (CPV) systems employ sunlight concentrated onto photovoltaic surfaces for the purpose of electricity generation. Thermoelectric, or "thermovoltaic" devices convert a temperature difference between dissimilar materials into an electric current.
Floating solar arrays
Floating solar arrays and solar canals are PV systems that float on--or are built over--the surface of drinking water reservoirs, quarry lakes, irrigation canals or remediation and tailing ponds. A small number of such systems exist in France, India, Japan, South Korea, the United Kingdom, Singapore, and the United States.[123][124][125][126][127] The systems are said to have advantages over photovoltaics on land. The cost of land is more expensive, and there are fewer rules and regulations for structures built on bodies of water not used for recreation. Unlike most land-based solar plants, floating arrays can be unobtrusive because they are hidden from public view. They achieve higher efficiencies than PV panels on land, because water cools the panels. The panels have a special coating to prevent rust or corrosion.[128] In May 2008, the Far Niente Winery in Oakville, California, pioneered the world's first floatovoltaic system by installing 994 solar PV modules with a total capacity of 477 kW onto 130 pontoons and floating them on the winery's irrigation pond.[129] Utility-scale floating PV farms are starting to be built. Kyocera will develop the world's largest, a 13.4 MW farm on the reservoir above Yamakura Dam in Chiba Prefecture[130] using 50,000 solar panels.[131][132] Salt-water resistant floating farms are also being constructed for ocean use.[133] The largest so far announced floatovoltaic project is a 350 MW power station in the Amazon region of Brazil.[134]
Perovskite solar cells
A perovskite solar cell (PSC) is a type of solar cell that includes a perovskite-structured compound, most commonly a hybrid organic–inorganic lead or tin halide-based material as the light-harvesting active layer.[135][136] Perovskite materials, such as methylammonium lead halides and all-inorganic cesium lead halide, are cheap to produce and simple to manufacture.
Solar-cell efficiencies of laboratory-scale devices using these materials have increased from 3.8% in 2009[137] to 25.7% in 2021 in single-junction architectures,[138][139] and, in silicon-based tandem cells, to 29.8%,[138][140] exceeding the maximum efficiency achieved in single-junction silicon solar cells. Perovskite solar cells have therefore been the fastest-advancing solar technology as of 2016.[135] With the potential of achieving even higher efficiencies and very low production costs, perovskite solar cells have become commercially attractive. Core problems and research subjects include their short- and long-term stability.[141]Solar-assisted heat pump
A heat pump is a device that provides heat energy from a source of heat to a destination called a "heat sink". Heat pumps are designed to move thermal energy opposite to the direction of spontaneous heat flow by absorbing heat from a cold space and releasing it to a warmer one. A solar-assisted heat pump represents the integration of a heat pump and thermal solar panels in a single integrated system. Typically these two technologies are used separately (or only placing them in parallel) to produce hot water.[142] In this system the solar thermal panel performs the function of the low temperature heat source and the heat produced is used to feed the heat pump's evaporator.[143] The goal of this system is to get high COP and then produce energy in a more efficient and less expensive way.
It is possible to use any type of solar thermal panel (sheet and tubes, roll-bond, heat pipe, thermal plates) or hybrid (mono/polycrystalline, thin film) in combination with the heat pump. The use of a hybrid panel is preferable because it allows covering a part of the electricity demand of the heat pump and reduces the power consumption and consequently the variable costs of the system.
Solar aircraft
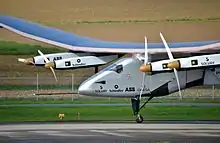
An electric aircraft is an aircraft that runs on electric motors rather than internal combustion engines, with electricity coming from fuel cells, solar cells, ultracapacitors, power beaming,[144] or batteries.
Currently, flying manned electric aircraft are mostly experimental demonstrators, though many small unmanned aerial vehicles are powered by batteries. Electrically powered model aircraft have been flown since the 1970s, with one report in 1957.[145][146] The first man-carrying electrically powered flights were made in 1973.[147] Between 2015 and 2016, a manned, solar-powered plane, Solar Impulse 2, completed a circumnavigation of the Earth.[148]
See also
- Heliostat
- List of solar energy topics
- Renewable heat
- Soil solarization
- Solar easement
- Solar energy use in rural Africa
- Solar updraft tower
- Solar power satellite
- Solar tracker
References
- "Solar Energy Perspectives: Executive Summary" (PDF). International Energy Agency. 2011. Archived from the original (PDF) on 13 January 2012.
- "Energy". Royal Society of Chemistry. 2 April 2014.
- "'Renewables' power ahead to become the world's cheapest source of energy in 2020". World Economic Forum. Retrieved 2022-01-25.
- "Levelized Cost Of Energy, Levelized Cost Of Storage, and Levelized Cost Of Hydrogen". Lazard.com. Retrieved 2022-01-25.
- "Saudi Arabia signed Power Purchase Agreement for 2,970MW Solar PV Projects". saudigulfprojects.com. Retrieved 2022-08-28.
- Smil (1991), p. 240
- "Natural Forcing of the Climate System". Intergovernmental Panel on Climate Change. Archived from the original on 29 September 2007. Retrieved 29 September 2007.
- Karuppu, Karthik; Sitaraman, Venk; NVICO (2019). Solar Assessment Guidance: A Guide for Solar Trainee, Trainer & Assessor Examination. Notion Press. ISBN 978-1646505227.
- "Radiation Budget". NASA Langley Research Center. 17 October 2006. Retrieved 29 September 2007.
- Somerville, Richard. "Historical Overview of Climate Change Science" (PDF). Intergovernmental Panel on Climate Change. Retrieved 29 September 2007.
- Vermass, Wim. "An Introduction to Photosynthesis and Its Applications". Arizona State University. Archived from the original on 3 December 1998. Retrieved 29 September 2007.
- Smil (2006), p. 12
- Morton, Oliver (6 September 2006). "Solar energy: A new day dawning?: Silicon Valley sunrise". Nature. 443 (7107): 19–22. Bibcode:2006Natur.443...19M. doi:10.1038/443019a. PMID 16957705. S2CID 13266273.
- Lewis, N. S.; Nocera, D. G. (2006). "Powering the Planet: Chemical challenges in solar energy utilization" (PDF). Proceedings of the National Academy of Sciences. 103 (43): 15729–35. Bibcode:2006PNAS..10315729L. doi:10.1073/pnas.0603395103. PMC 1635072. PMID 17043226. Retrieved 7 August 2008.
- "Energy conversion by photosynthetic organisms". Food and Agriculture Organization of the United Nations. Retrieved 25 May 2008.
- Archer, Cristina; Jacobson, Mark. "Evaluation of Global Wind Power". Stanford. Retrieved 3 June 2008.
- "Renewable Energy Sources" (PDF). Renewable and Appropriate Energy Laboratory. p. 12. Archived from the original (PDF) on 19 November 2012. Retrieved 6 December 2012.
- "Total Primary Energy Consumption". Energy Information Administration. Retrieved 28 June 2022.
- "Total Electricity Net Consumption". Energy Information Administration. Retrieved 28 June 2022.
- Bond, Kingsmill (April 2021). "The sky's the limit" (PDF). epbr. Carbon Tracker Initiative. p. 6. Archived (PDF) from the original on April 30, 2021. Retrieved October 22, 2021.
- "Energy and the challenge of sustainability" (PDF). United Nations Development Programme and World Energy Council. September 2000. Retrieved 17 January 2017.
- Philibert, Cédric (2005). "The Present and Future use of Solar Thermal Energy as a Primary Source of Energy" (PDF). IEA. Archived (PDF) from the original on 26 April 2012.
- "Solar Energy Technologies and Applications". Canadian Renewable Energy Network. Archived from the original on 25 June 2002. Retrieved 22 October 2007.
- Frank Kryza (2003). The Power of Light. McGraw Hill Professional. pp. 64, 135. ISBN 978-0-07-140021-3. Retrieved 30 August 2022.
- Smith, Zachary Alden; Taylor, Katrina D. (2008). Renewable And Alternative Energy Resources: A Reference Handbook. ABC-CLIO. p. 174. ISBN 978-1-59884-089-6.
- "American Inventor Uses Egypt's Sun for Power – Appliance Concentrates the Heat Rays and Produces Steam, Which Can Be Used to Drive Irrigation Pumps in Hot Climates" (PDF). The New York Times. 2 July 1916.
- "Renewables for Heating and Cooling" (PDF). International Energy Agency. Archived from the original (PDF) on 24 September 2015. Retrieved 13 August 2015.
- Weiss, Werner; Bergmann, Irene; Faninger, Gerhard. "Solar Heat Worldwide (Markets and Contributions to the Energy Supply 2005)" (PDF). International Energy Agency. Retrieved 30 May 2008.
- "Solar District Heating In The People's Republic of China" (PDF). Status and Development Potential. Asian Development Bank: 23. 1 July 2019. Retrieved 6 July 2021.
- Del Chiaro, Bernadette; Telleen-Lawton, Timothy. "Solar Water Heating (How California Can Reduce Its Dependence on Natural Gas)" (PDF). Environment California Research and Policy Center. Archived from the original (PDF) on 27 September 2007. Retrieved 29 September 2007.
- Apte, J.; et al. "Future Advanced Windows for Zero-Energy Homes" (PDF). American Society of Heating, Refrigerating and Air-Conditioning Engineers. Archived from the original (PDF) on 10 April 2008. Retrieved 9 April 2008.
- "Energy Consumption Characteristics of Commercial Building HVAC Systems Volume III: Energy Savings Potential" (PDF). United States Department of Energy. Retrieved 24 June 2008.
- Mazria (1979), pp. 29–35
- Bright, David (18 February 1977). "Passive solar heating simpler for the average owner". Bangor Daily News. Retrieved 3 July 2011.
- Mazria (1979), p. 255
- Balcomb (1992), p. 56
- Balcomb (1992), p. 57
- Anderson and Palkovic (1994), p. xi
- Butti and Perlin (1981), pp. 54–59
- , Anderson and Palkovic (1994), p. xii
- Anderson and Palkovic (1994), p. xiii
- Stine, W.B. & Harrigan, R.W. (1982). "Shenandoah Solar Total Energy Project". NASA Sti/Recon Technical Report N. John Wiley. 83: 25168. Bibcode:1982STIN...8325168L. Retrieved 20 July 2008.
- Bartlett (1998), pp. 393–94
- Thomson-Philbrook, Julia. "Right to Dry Legislation in New England and Other States". Connecticut General Assembly. Retrieved 27 May 2008.
- "Solar Buildings (Transpired Air Collectors – Ventilation Preheating)" (PDF). National Renewable Energy Laboratory. Retrieved 29 September 2007.
- Leon (2006), p. 62
- Tiwari (2003), pp. 368–71
- Daniels (1964), p. 6
- "SODIS solar water disinfection". EAWAG (The Swiss Federal Institute for Environmental Science and Technology). Retrieved 2 May 2008.
- "Household Water Treatment Options in Developing Countries: Solar Disinfection (SODIS)" (PDF). Centers for Disease Control and Prevention. Archived from the original (PDF) on 29 May 2008. Retrieved 13 May 2008.
- "Household Water Treatment and Safe Storage". World Health Organization. Archived from the original on October 25, 2004. Retrieved 2 May 2008.
- Shilton A.N.; Powell N.; Mara D.D.; Craggs R. (2008). "Solar-powered aeration and disinfection, anaerobic co-digestion, biological CO(2) scrubbing and biofuel production: the energy and carbon management opportunities of waste stabilization ponds". Water Sci. Technol. 58 (1): 253–58. doi:10.2166/wst.2008.666. PMID 18653962.
- Tadesse I.; Isoaho S.A.; Green F.B.; Puhakka J.A. (2003). "Removal of organics and nutrients from tannery effluent by advanced integrated Wastewater Pond Systems technology". Water Sci. Technol. 48 (2): 307–14. doi:10.2166/wst.2003.0135. PMID 14510225.
- Mancini, Tom (10 January 2006). "Advantages of Using Molten Salt". Sandia National Laboratories. Archived from the original on 5 June 2011. Retrieved 14 July 2011.
- Molten salt energy storage system – A feasibility study Jones, B.G.; Roy, R.P.; Bohl, R.W. (1977) – Smithsonian/NASA ADS Physics Abstract Service. Abstract accessed December 2007
- Biello, David. "How to Use Solar Energy at Night". Scientific American. Retrieved 19 June 2011.
- Ehrlich, Robert, 2013, "Renewable Energy: A First Course," CRC Press, Chap. 13.1.22 Thermal storage p. 375 ISBN 978-1-4398-6115-8
- Parabolic Trough Thermal Energy Storage Technology Archived 1 September 2013 at the Wayback Machine Parabolic Trough Solar Power Network. 4 April 2007. Accessed December 2007
- Here comes the sun Chile greenlights enormous 400-megawatt solar project www.thisischile.cl Friday, 23 August 2013 retrieved 30 August 2013
- "Energy Sources: Solar". Department of Energy. Archived from the original on 14 April 2011. Retrieved 19 April 2011.
- "Solar is now 'cheapest electricity in history', confirms IEA". 13 October 2020.
- "Global Electricity Review 2022". Ember. 2022-03-29. Retrieved 2022-04-03.
- "Levelized Cost Of Energy, Levelized Cost Of Storage, and Levelized Cost Of Hydrogen". Lazard.com. Retrieved 2022-04-03.
- "Net Zero by 2050 – Analysis". IEA. Retrieved 2021-10-14.
- Lo Piano, Samuele; Mayumi, Kozo (2017). "Toward an integrated assessment of the performance of photovoltaic systems for electricity generation". Applied Energy. 186 (2): 167–74. doi:10.1016/j.apenergy.2016.05.102. S2CID 156783885.
- Bazilian, M.; Onyeji, I.; Liebreich, M.; MacGill, I.; Chase, J.; Shah, J.; Gielen, D.; Arent, D.; Landfear, D.; Zhengrong, S. (2013). "Re-considering the economics of photovoltaic power" (PDF). Renewable Energy. 53: 329–338. CiteSeerX 10.1.1.692.1880. doi:10.1016/j.renene.2012.11.029. Archived from the original (PDF) on 31 May 2014. Retrieved 4 September 2015.
- Palz, Wolfgang (2013). Solar Power for the World: What You Wanted to Know about Photovoltaics. CRC Press. pp. 131–. ISBN 978-981-4411-87-5.
- "Why did renewables become so cheap so fast?". Our World in Data. December 1, 2020.
- Shubbak, Mahmood H. (2019). "The technological system of production and innovation: The case of photovoltaic technology in China". Research Policy. 48 (4): 993–1015. doi:10.1016/j.respol.2018.10.003. S2CID 158742469.
- Renewable Energy Policy Network for the 21st century (REN21), Renewables 2010 Global Status Report, Paris, 2010, pp. 1–80.
- "PHOTOVOLTAICS REPORT" (PDF). Fraunhofer Institute for Solar Energy Systems. 16 September 2020. p. 4.
- "Renewables 2019". IEA. Retrieved 26 January 2020.
- "KAHRAMAA and Siraj Energy Sign Agreements for Al-Kharsaah Solar PV Power Plant". Qatar General Electricity & Water Corporation "KAHRAMAA". 20 January 2020. Retrieved 26 January 2020.
- Sunil Prasad Lohani, Andrew Blakers: 100% renewable energy with pumped-hydro-energy storage in Nepal. In: Clean Energy 5, 2, 2021, 243–253, doi:10.1093/ce/zkab011.
- Martin and Goswami (2005), p. 45
- "Darmstadt University of Technology solar decathlon home design". Darmstadt University of Technology. Archived from the original on 18 October 2007. Retrieved 25 April 2008.
- Schittich (2003), p. 14
- Butti and Perlin (1981), pp. 4, 159
- Balcomb (1992)
- Rosenfeld, Arthur; et al. "Painting the Town White – and Green". Heat Island Group. Archived from the original on 14 July 2007. Retrieved 29 September 2007.
- Jeffrey C. Silvertooth. "Row Spacing, Plant Population, and Yield Relationships". University of Arizona. Retrieved 24 June 2008.
- Kaul (2005), pp. 169–74
- Butti and Perlin (1981), pp. 42–46
- Bénard (1981), p. 347
- "A Powerhouse Winery". News Update. Novus Vinum. 27 October 2008. Retrieved 5 November 2008.
- Butti and Perlin (1981), p. 19
- Butti and Perlin (1981), p. 41
- "The World Solar Challenge – The Background" (PDF). Australian and New Zealand Solar Energy Society. Archived from the original (PDF) on 19 July 2008. Retrieved 5 August 2008.
- "North American Solar Challenge". New Resources Group. Retrieved 3 July 2008.
- "South African Solar Challenge". Advanced Energy Foundation. Archived from the original on 12 June 2008. Retrieved 3 July 2008.
- Vehicle auxiliary power applications for solar cells. 1991. pp. 187–191. ISBN 0-85296-525-7. Retrieved 11 October 2008.
- "Systaic AG: Demand for Car Solar Roofs Skyrockets". Archived from the original on 5 May 2009. Retrieved 29 March 2011.
- Electrical Review Vol. 201, No. 7, 12 August 1977
- Schmidt, Theodor. "Solar Ships for the new Millennium". TO Engineering. Archived from the original on 9 October 2007. Retrieved 30 September 2007.
- "The sun21 completes the first transatlantic crossing with a solar powered boat". Transatlantic 21. Retrieved 30 September 2007.
- "PlanetSolar, the first solar-powered round-the-world voyage". PlanetSolar. 14 August 2015. Retrieved 20 November 2016.
- "EVWORLD FEATURE: Sunseeker Seeks New Records:SUNSEEKER | SAILPLANE | SOARING | GLIDER | ELECTRIC | RAYMOND | PV | PHOTOVOLTAIC | SOLAR | SUN | PLANE | AIRCRAFT | KITTYHAWK | AC PROPULSION". Archived from the original on 8 February 2008. Retrieved 8 February 2008.
- "Solar-Power Research and Dryden". NASA. Retrieved 30 April 2008.
- "The NASA ERAST HALE UAV Program". Greg Goebel. Archived from the original on 10 February 2008. Retrieved 30 April 2008.
- Solar Impulse Project. "HB-SIA Mission". Archived from the original on 26 July 2011. Retrieved 5 December 2009.
- "Phenomena which affect a solar balloon". pagesperso-orange.fr. Retrieved 19 August 2008.
- Bolton (1977), p. 1
- Wasielewski MR Photoinduced electron transfer in supramolecular systems for artificial photosynthesis. Chem. Rev. 1992; 92: 435–61.
- Hammarstrom L. and Hammes-Schiffer S. Artificial Photosynthesis and Solar Fuels. Accounts of Chemical Research 2009; 42 (12): 1859–60.
- Gray H.B. Powering the planet with solar fuel. Nature Chemistry 2009; 1: 7.
- Agrafiotis (2005), p. 409
- Zedtwitz (2006), p. 1333
- "Solar Energy Project at the Weizmann Institute Promises to Advance the use of Hydrogen Fuel". Weizmann Institute of Science. Archived from the original on 6 April 2008. Retrieved 25 June 2008.
- Balcomb(1992), p. 6
- "Request for Participation Summer 2005 Demand Shifting with Thermal Mass" (PDF). Demand Response Research Center. Archived from the original (PDF) on 7 September 2008. Retrieved 26 November 2007.
- Butti and Perlin (1981), pp. 212–14
- "Advantages of Using Molten Salt". Sandia National Laboratory. Retrieved 29 September 2007.
- "PV Systems and Net Metering". Department of Energy. Archived from the original on 4 July 2008. Retrieved 31 July 2008.
- "Pumped Hydro Storage". Electricity Storage Association. Archived from the original on 21 June 2008. Retrieved 31 July 2008.
- Butti and Perlin (1981), pp. 63, 77, 101
- Butti and Perlin (1981), p. 249
- Yergin (1991), pp. 634, 653–73
- "Chronicle of Fraunhofer-Gesellschaft". Fraunhofer-Gesellschaft. Retrieved 4 November 2007.
- Butti, and Perlin (1981), p. 117
- Butti and Perlin (1981), p. 139
- Weiss, Werner; Bergmann, Irene; Faninger, Gerhard. "Solar Heat Worldwide – Markets and Contribution to the Energy Supply 2006" (PDF). International Energy Agency. Retrieved 9 June 2008.
- "IEA Says Solar May Provide a Third of Global Energy by 2060". Bloomberg Businessweek. 1 December 2011.
- "Kyocera, partners announce construction of the world's largest floating solar PV Plant in Hyogo prefecture, Japan". SolarServer.com. 4 September 2014. Archived from the original on 24 September 2015. Retrieved 11 June 2016.
- "Running Out of Precious Land? Floating Solar PV Systems May Be a Solution". EnergyWorld.com. 7 November 2013. Archived from the original on 26 December 2014. Retrieved 11 June 2016.
- "Vikram Solar commissions India's first floating PV plant". SolarServer.com. 13 January 2015. Archived from the original on 2 March 2015.
- "Sunflower Floating Solar Power Plant In Korea". CleanTechnica. 21 December 2014. Archived from the original on 15 May 2016. Retrieved 11 June 2016.
- "Short Of Land, Singapore Opts For Floating Solar Power Systems". CleanTechnica. 5 May 2014. Archived from the original on 14 March 2016. Retrieved 11 June 2016.
- Erica Goodemay, New Solar Plants Generate Floating Green Power Archived 27 May 2016 at the Wayback Machine, New York Times, 20 May 2016.
- "Winery goes solar with Floatovoltaics". SFGate. 29 May 2008. Archived from the original on 7 May 2013. Retrieved 31 May 2013.
- "Yamakura Dam in Chiba Prefecture". The Japan Dam Foundation. Archived from the original on 2 February 2015. Retrieved 1 February 2015.
- Kyocera and Century Tokyo Leasing to Develop 13.4MW Floating Solar Power Plant on Reservoir in Chiba Prefecture, Japan Archived 25 June 2016 at the Wayback Machine, Kyocera, 22 December 2014
- New Solar Plants Generate Floating Green Power Archived 28 December 2016 at the Wayback Machine NYT 20 May 2016
- Solar Panels Floating on Water Could Power Japan's Homes Archived 11 June 2016 at the Wayback Machine, National Geographic, Bryan Lufkin, 16 January 2015
- Upadhyay, Anand (6 April 2015). "Brazil Announces Huge 350 MW Floating Solar Power Plant". CleanTechnica.com. Archived from the original on 18 May 2015. Retrieved 11 June 2016.
- Manser, Joseph S.; Christians, Jeffrey A.; Kamat, Prashant V. (2016). "Intriguing Optoelectronic Properties of Metal Halide Perovskites". Chemical Reviews. 116 (21): 12956–13008. doi:10.1021/acs.chemrev.6b00136. PMID 27327168.
- Hamers, Laurel (26 July 2017). "Perovskites power up the solar industry". Science News.
- Kojima, Akihiro; Teshima, Kenjiro; Shirai, Yasuo; Miyasaka, Tsutomu (May 6, 2009). "Organometal Halide Perovskites as Visible-Light Sensitizers for Photovoltaic Cells". Journal of the American Chemical Society. 131 (17): 6050–6051. doi:10.1021/ja809598r. PMID 19366264.
- "Best Research-Cell Efficiencies" (PDF). National Renewable Energy Laboratory. 2022-06-30. Retrieved 2022-07-12.
- Min, Hanul; Lee, Do Yoon; Kim, Junu; Kim, Gwisu; Lee, Kyoung Su; Kim, Jongbeom; Paik, Min Jae; Kim, Young Ki; Kim, Kwang S.; Kim, Min Gyu; Shin, Tae Joo; Il Seok, Sang (21 October 2021). "Perovskite solar cells with atomically coherent interlayers on SnO2 electrodes". Nature. 598 (7881): 444–450. doi:10.1038/s41586-021-03964-8. PMID 34671136. S2CID 239052065.
- Helmholtz-Zentrum Berlin für Materialien und Energie. "World record again at HZB: Almost 30 % efficiency for next-generation tandem solar cells". HZB Website.
- Sun, Kai; Wang, Yanyan; Xu, Haoyuan; Zhang, Jing; Zhu, Yuejin; Hu, Ziyang (2019). "Short-Term Stability of Perovskite Solar Cells Affected by In Situ Interface Modification". Solar RRL. 3 (9): 1900089. doi:10.1002/solr.201900089. S2CID 202229877.
- "Solar-assisted heat pumps". Archived from the original on 28 February 2020. Retrieved 21 June 2016.
- "Pompe di calore elio-assistite" (in Italian). Archived from the original on 7 January 2012. Retrieved 21 June 2016.
- "Power Beaming". Archived from the original on February 17, 2013.
- Noth, André (July 2008). "History of Solar Flight" (PDF). Autonomous Systems Lab. Zürich: Swiss Federal Institute of Technology. p. 3. Archived from the original (PDF) on 1 February 2012. Retrieved 8 July 2010.
Günter Rochelt was the designer and builder of Solair I, a 16 m wingspan solar airplane ... 21st of August 1983 he flew in Solair I, mostly on solar energy and also thermals, during 5 hours 41 minutes.
- "Infographic: A Timeline Of The Present And Future Of Electric Flight". Popular Science. 20 August 2015. Archived from the original on 14 January 2016. Retrieved 7 January 2016.
- Taylor, John W R (1974). Jane's All the World's Aircraft 1974-75. London: Jane's Yearbooks. p. 573. ISBN 0-354-00502-2.
- Batrawy, Aya (9 March 2015). "Solar-powered plane takes off for flight around the world". Associated Press. Archived from the original on 6 March 2016. Retrieved 14 March 2015.
Further reading

Library resources about Solar energy |
- Denzer, Anthony (2013). The Solar House: Pioneering Sustainable Design. Rizzoli. ISBN 978-0-8478-4005-2. Archived from the original on 26 July 2013.