Human impact on marine life
Part of a series of overviews on |
Marine life |
---|
![]() |
|
![]() |
Human activities affect marine life and marine habitats through overfishing, habitat loss, the introduction of invasive species, ocean pollution, ocean acidification and ocean warming. These impact marine ecosystems and food webs and may result in consequences as yet unrecognised for the biodiversity and continuation of marine life forms.[3]
According to the IPCC (2019), since 1950 "many marine species across various groups have undergone shifts in geographical range and seasonal activities in response to ocean warming, sea ice change and biogeochemical changes, such as oxygen loss, to their habitats."[4]
It has been estimated only 13% of the ocean area remains as wilderness, mostly in open ocean areas rather than along the coast.[5]
Overfishing
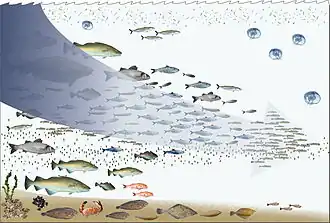
Overfishing of high trophic fish like tuna can result in
them being replaced by low trophic organisms, like jellyfish
Overfishing is occurring in one third of world fish stocks, according to a 2018 report by the Food and Agriculture Organization of the United Nations.[6] In addition, industry observers believe illegal, unreported and unregulated fishing occurs in most fisheries, and accounts for up to 30% of total catches in some important fisheries.[7] In a phenomenon called fishing down the foodweb, the mean trophic level of world fisheries has declined because of overfishing high trophic level fish.[8]
"It is almost as though we use our military to fight the animals in the ocean. We are gradually winning this war to exterminate them."
– Daniel Pauly, pioneer on human impacts on global fisheries, [9]
Habitat loss
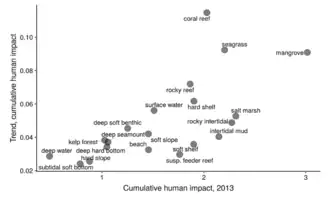
Coastal ecosystems are being particularly damaged by humans.[10] Significant habitat loss is occurring particularly in seagrass meadows, mangrove forests and coral reefs, all of which are in global decline due to human disturbances.
Coral reefs are among the more productive and diverse ecosystems on the planet, but one-fifth of them have been lost in recent years due to anthropogenic disturbances.[11][12] Coral reefs are microbially driven ecosystems that rely on marine microorganisms to retain and recycle nutrients in order to thrive in oligotrophic waters. However, these same microorganisms can also trigger feedback loops that intensify declines in coral reefs, with cascading effects across biogeochemical cycles and marine food webs. A better understanding is needed of the complex microbial interactions within coral reefs if reef conservation has a chance of success in the future.[13]
Seagrass meadows have lost 30,000 km2 (12,000 sq mi) during recent decades. Seagrass ecosystem services, currently worth about $US1.9 trillion per year, include nutrient cycling, the provision of food and habitats for many marine animals, including the endangered dugongs, manatee and green turtles, and major facilitations for coral reef fish.[10]
One-fifth of the world's mangrove forests have also been lost since 1980.[14] The most pressing threat to kelp forests may be the overfishing of coastal ecosystems, which by removing higher trophic levels facilitates their shift to depauperate urchin barrens.[15]
Invasive species

An invasive species is a species not native to a particular location which can spread to a degree that causes damage to the environment, human economy or human health.[16] In 2008, Molnar et al. documented the pathways of hundreds of marine invasive species and found shipping was the dominant mechanism for the transfer of invasive species in the ocean. The two main maritime mechanisms of transporting marine organisms to other ocean environments are via hull fouling and the transfer of ballast water.[17]
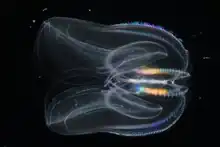
Ballast water taken up at sea and released in port is a major source of unwanted exotic marine life. The invasive freshwater zebra mussels, native to the Black, Caspian, and Azov seas, were probably transported to the Great Lakes via ballast water from a transoceanic vessel.[18] Meinesz believes that one of the worst cases of a single invasive species causing harm to an ecosystem can be attributed to a seemingly harmless jellyfish. Mnemiopsis leidyi, a species of comb jellyfish that spread so it now inhabits estuaries in many parts of the world, was first introduced in 1982, and thought to have been transported to the Black Sea in a ship's ballast water. The population of the jellyfish grew exponentially and, by 1988, it was wreaking havoc upon the local fishing industry. "The anchovy catch fell from 204,000 tons in 1984 to 200 tons in 1993; sprat from 24,600 tons in 1984 to 12,000 tons in 1993; horse mackerel from 4,000 tons in 1984 to zero in 1993."[19] Now that the jellyfish have exhausted the zooplankton, including fish larvae, their numbers have fallen dramatically, yet they continue to maintain a stranglehold on the ecosystem.
Invasive species can take over once occupied areas, facilitate the spread of new diseases, introduce new genetic material, alter underwater seascapes, and jeopardize the ability of native species to obtain food. Invasive species are responsible for about $138 billion annually in lost revenue and management costs in the US alone.[20]
Marine pollution
Marine pollution occurs when substances used or spread by humans, such as industrial, agricultural and residential waste, particles, noise, excess carbon dioxide or invasive organisms enter the ocean and cause harmful effects there. The majority of this waste (80%) comes from land-based activity, although marine transportation significantly contributes as well.[21] Since most inputs come from land, either via the rivers, sewage or the atmosphere, it means that continental shelves are more vulnerable to pollution. Air pollution is also a contributing factor by carrying off iron, carbonic acid, nitrogen, silicon, sulfur, pesticides or dust particles into the ocean.[22] The pollution often comes from nonpoint sources such as agricultural runoff, wind-blown debris, and dust. These nonpoint sources are largely due to runoff that enters the ocean through rivers, but wind-blown debris and dust can also play a role, as these pollutants can settle into waterways and oceans.[23] Pathways of pollution include direct discharge, land runoff, ship pollution, atmospheric pollution and, potentially, deep sea mining.
The types of marine pollution can be grouped as pollution from marine debris, plastic pollution, including microplastics, ocean acidification, nutrient pollution, toxins and underwater noise. Plastic pollution in the ocean is a type of marine pollution by plastics, ranging in size from large original material such as bottles and bags, down to microplastics formed from the fragmentation of plastic material. Marine debris is mainly discarded human rubbish which floats on, or is suspended in the ocean. Plastic pollution is harmful to marine life.
Another concern is the runoff of nutrients (nitrogen and phosphorus) from intensive agriculture, and the disposal of untreated or partially treated sewage to rivers and subsequently oceans. These nitrogen and phosphorus nutrients (which are also contained in fertilizers) stimulate phytoplankton and macroalgal growth, which can lead to harmful algal blooms (eutrophication) which can be harmful to humans as well as marine creatures. Excessive algal growth can also smother sensitive coral reefs and lead to loss of biodiversity and coral health. A second major concern is that the degradation of algal blooms can lead to consumption of oxygen in coastal waters, a situation that may worsen with climate change as warming reduces vertical mixing of the water column.[24]Nutrient pollution
Nutrient pollution is a primary cause of eutrophication of surface waters, in which excess nutrients, usually nitrates or phosphates, stimulate algae growth. This algae then dies, sinks, and is decomposed by bacteria in the water. This decomposition process consumes oxygen, depleting the supply for other marine life and creating what is referred to as a "dead zone." Dead zones are hypoxic, meaning the water has very low levels of dissolved oxygen. This kills off marine life or forces it to leave the area, removing life from the area and giving it the name dead zone. Hypoxic zones or dead zones can occur naturally, but nutrient pollution from human activity has turned this natural process into an environmental problem.[25]
There are five main sources of nutrient pollution. The most common source of nutrient runoff is municipal sewage. This sewage can reach waterways through storm water, leaks, or direct dumping of human sewage into bodies of water. The next biggest sources come from agricultural practices. Chemical fertilizers used in farming can seep into ground water or be washed away in rainwater, entering water ways and introducing excess nitrogen and phosphorus to these environments. Livestock waste can also enter waterways and introduce excess nutrients. Nutrient pollution from animal manure is most intense from industrial animal agriculture operations, in which hundreds or thousands of animals are raised in one concentrated area. Stormwater drainage is another source of nutrient pollution. Nutrients and fertilizers from residential properties and impervious surfaces can be picked up in stormwater, which then runs into nearby rivers and streams that eventually lead to the ocean. The fifth main source of nutrient runoff is aquaculture, in which aquatic organisms are cultivated under controlled conditions. The excrement, excess food, and other organic wastes created by these operations introduces excess nutrients into the surrounding water.[26]
Toxic chemicals
Toxic chemicals can adhere to tiny particles which are then taken up by plankton and benthic animals, most of which are either deposit feeders or filter feeders. In this way, toxins are concentrated upward within ocean food chains. Many particles combine chemically in a manner which depletes oxygen, causing estuaries to become anoxic. Pesticides and toxic metals are similarly incorporated into marine food webs, harming the biological health of marine life. Many animal feeds have a high fish meal or fish hydrolysate content. In this way, marine toxins are transferred back to farmed land animals, and then to humans.
Phytoplankton concentrations have increased over the last century in coastal waters, and more recently have declined in the open ocean. Increases in nutrient runoff from land may explain the rise in coastal phytoplankton, while warming surface temperatures in the open ocean may have strengthened stratification in the water column, reducing the flow of nutrients from the deep that open ocean phytoplankton find useful.[27]
Plastic pollution
Over 300 million tons of plastic are produced every year, half of which are used in single-use products like cups, bags, and packaging. At least 14 million[28] tons of plastic enter the oceans every year. It is impossible to know for sure, but it is estimated that about 150 million metric tons of plastic exists in our oceans. Plastic pollution makes up 80% of all marine debris from surface waters to deep-sea sediments. Because plastics are light, much of this pollution is seen in and around the ocean surface, but plastic trash and particles are now found in most marine and terrestrial habitats, including the deep sea, Great Lakes, coral reefs, beaches, rivers, and estuaries. The most eye-catching evidence of the ocean plastic problem are the garbage patches that accumulate in gyre regions. A gyre is a circular ocean current formed by the Earth's wind patterns and the forces created by the rotation of the planet.[29] There are five main ocean gyres: the North and South Pacific Subtropical Gyres, the North and South Atlantic Subtropical Gyres, and the Indian Ocean Subtropical Gyre. There are significant garbage patches in each of these.[30]
Larger plastic waste can be ingested by marine species, filling their stomachs and leading them to believe they are full when in fact they have taken in nothing of nutritional value. This can bring seabirds, whales, fish, and turtles to die of starvation with plastic-filled stomachs. Marine species can also be suffocated or entangled in plastic garbage.[31]
The biggest threat of ocean plastic pollution comes from microplastics. These are small fragments of plastic debris, some of which were produced to be this small such as microbeads. Other microplastics come from the weathering of larger plastic waste. Once larger pieces of plastic waste enter the ocean, or any waterway, the sunlight exposure, temperature, humidity, waves, and wind begin to break the plastic down into pieces smaller than five millimeters long. Plastics can also be broken down by smaller organisms who will eat plastic debris, breaking it down into small pieces, and either excrete these microplastics or spit them out. In lab tests, it was found that amphipods of the species Orchestia gammarellus could quickly devour pieces of plastic bags, shredding a single bag into 1.75 million microscopic fragments.[32] Although the plastic is broken down, it is still a man-made material that does not biodegrade. It is estimated that approximately 90% of the plastics in the pelagic marine environment are microplastics.[29] These microplastics are frequently consumed by marine organisms at the base of the food chain, like plankton and fish larvae, which leads to a concentration of ingested plastic up the food chain. Plastics are produced with toxic chemicals which then enter the marine food chain, including the fish that some humans eat.[33]
- Microplastics among sand and glass spheres in sediment from the Rhine. The white bar represents 1 mm.
- Vast plastic garbage patches have accumulated at the centre of ocean gyres.[34]
- Model results for the count density of small planktonic plastic particles.[35]
Red: more denseGreen: less dense - Interactions between marine microorganisms and microplastics [36]
Noise pollution
There is a natural soundscape to the ocean that organisms have evolved around for tens of thousands of years. However, human activity has disrupted this soundscape, largely drowning out sounds organisms depend on for mating, warding off predators, and travel. Ship and boat propellers and engines, industrial fishing, coastal construction, oil drilling, seismic surveys, warfare, sea-bed mining and sonar-based navigation have all introduced noise pollution to ocean environments. Shipping alone has contributed an estimated 32-fold increase of low-frequency noise along major shipping routes in the past 50 years, driving marine animals away from vital breeding and feeding grounds.[37] Sound is the sensory cue that travels the farthest through the ocean, and anthropogenic noise pollution disrupts organisms' ability to utilize sound. This creates stress for the organisms that can affect their overall health, disrupting their behavior, physiology, and reproduction, and even causing mortality.[38] Sound blasts from seismic surveys can damage the ears of marine animals and cause serious injury. Noise pollution is especially damaging for marine mammals that rely on echolocation, such as whales and dolphins. These animals use echolocation to communicate, navigate, feed, and find mates, but excess sound interferes with their ability to use echolocation and, therefore, perform these vital tasks.[39]
Mining
The prospect of deep sea mining has led to concerns from scientists and environmental groups over the impacts on fragile deep sea ecosystems and wider impacts on the ocean's biological pump.[40][41]
Human induced disease
Rapid change to ocean environments allows disease to flourish. Disease-causing microbes can change and adapt to new ocean conditions much more quickly than other marine life, giving them an advantage in ocean ecosystems. This group of organisms includes viruses, bacteria, fungi, and protozoans. While these pathogenic organisms can quickly adapt, other marine life is weakened by rapid changes to their environment. In addition, microbes are becoming more abundant due to aquaculture, the farming of aquatic life, and human waste polluting the ocean. These practices introduce new pathogens and excess nutrients into the ocean, further encouraging the survival of microbes.[42]
Some of these microbes have wide host ranges and are referred to as multi-host pathogens. This means that the pathogen can infect, multiply, and be transmitted from different, unrelated species. Multi-host pathogens are especially dangerous because they can infect many organisms, but may not be deadly to all them. This means the microbes can exist in species that are more resistant and use these organisms as vessels for continuously infecting a susceptible species. In this case, the pathogen can completely wipe out the susceptible species while maintaining a supply of host organisms.[42]
Climate change
In marine environments, microbial primary production contributes substantially to CO2 sequestration. Marine microorganisms also recycle nutrients for use in the marine food web and in the process release CO2 to the atmosphere. Microbial biomass and other organic matter (remnants of plants and animals) are converted to fossil fuels over millions of years. By contrast, burning of fossil fuels liberates greenhouse gases in a small fraction of that time. As a result, the carbon cycle is out of balance, and atmospheric CO2 levels will continue to rise as long as fossil fuels continue to be burnt.[43]
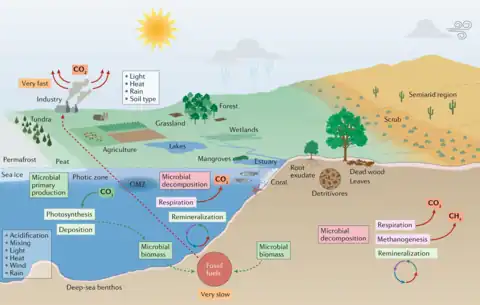
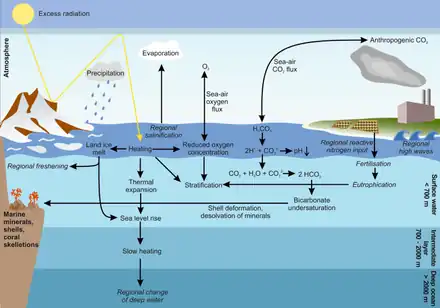
Ocean warming
.gif)
Source: NASA GISS
Most heat energy from global warming goes into the ocean, and not into the atmosphere or warming up the land.[45][46] Scientists realized over 30 years ago the ocean was a key fingerprint of human impact on climate change and "the best opportunity for major improvement in our understanding of climate sensitivity is probably monitoring of internal ocean temperature".[47]
Marine organisms are moving to cooler parts of the ocean as global warming proceeds. For example, a group of 105 marine fish and invertebrate species were monitored along the US Northeast coast and in the eastern Bering Sea. During the period from 1982 to 2015, the average center of biomass for the group shifted northward about 10 miles as well moving about 20 feet deeper.[48][49]

There is evidence increasing ocean temperatures are taking a toll on marine ecosystem. For example, a study on phytoplankton changes in the Indian Ocean indicates a decline of up to 20% in marine phytoplankton during the past six decades.[51] During summer, the western Indian Ocean is home to one of the largest concentrations of marine phytoplankton blooms in the world. Increased warming in the Indian Ocean enhances ocean stratification, which prevents nutrient mixing in the euphotic zone where ample light is available for photosynthesis. Thus, primary production is constrained and the region's entire food web is disrupted. If rapid warming continues, the Indian Ocean could transform into an ecological desert and cease being productive.[51]
The Antarctic oscillation (also called the Southern Annular Mode) is a belt of westerly winds or low pressure surrounding Antarctica which moves north or south according to which phase it is in.[54] In its positive phase, the westerly wind belt that drives the Antarctic Circumpolar Current intensifies and contracts towards Antarctica,[55] while its negative phase the belt moves towards the Equator. Winds associated with the Antarctic oscillation cause oceanic upwelling of warm circumpolar deep water along the Antarctic continental shelf.[56][57] This has been linked to ice shelf basal melt,[58] representing a possible wind-driven mechanism that could destabilize large portions of the Antarctic Ice Sheet.[59] The Antarctic oscillation is currently in the most extreme positive phase that has occurred for over a thousand years. Recently this positive phase has been further intensifying, and this has been attributed to increasing greenhouse gas levels and later stratospheric ozone depletion.[60][61] These large-scale alterations in the physical environment are "driving change through all levels of Antarctic marine food webs".[52][53] Ocean warming is also changing the distribution of Antarctic krill.[52][53] Antarctic krill is the keystone species of the Antarctic ecosystem beyond the coastal shelf, and is an important food source for marine mammals and birds.[62]
The IPCC (2019) says marine organisms are being affected globally by ocean warming with direct impacts on human communities, fisheries, and food production.[63] It is likely there will be a 15% decrease in the number of marine animals and a decrease of 21% to 24% in fisheries catches by the end of the 21st century because of climate change.[64]
A 2020 study reports that by 2050 global warming could be spreading in the deep ocean seven times faster than it is now, even if emissions of greenhouse gases are cut. Warming in mesopelagic and deeper layers could have major consequences for the deep ocean food web, since ocean species will need to move to stay at survival temperatures.[65][66]
Rising sea levels
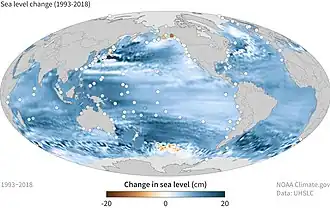
Coastal ecosystems are facing further changes because of rising sea levels. Some ecosystems can move inland with the high-water mark, but others are prevented from migrating due to natural or artificial barriers. This coastal narrowing, called coastal squeeze if human-made barriers are involved, can result in the loss of habitats such as mudflats and marshes.[68][69] Mangroves and tidal marshes adjust to rising sea levels by building vertically using accumulated sediment and organic matter. If sea level rise is too rapid, they will not be able to keep up and will instead be submerged.[70]
- As the sea level rises it moves further inland
Coral, important for bird and fish life, also needs to grow vertically to remain close to the sea surface in order to get enough energy from sunlight. So far it has been able to keep up, but might not be able to do so in the future.[73] These ecosystems protect against storm surges, waves and tsunamis. Losing them makes the effects of sea level rise worse.[74][75] Human activities, such as dam building, can prevent natural adaptation processes by restricting sediment supplies to wetlands, resulting in the loss of tidal marshes.[76] When seawater moves inland, the coastal flooding can cause problems with existing terrestrial ecosystems, such as contaminating their soils.[77] The Bramble Cay melomys is the first known land mammal to go extinct as a result of sea level rise.[78][79]
Ocean circulation and salinity
Ocean salinity is a measure of how much dissolved salt is in the ocean. The salts come from erosion and transport of dissolved salts from the land. The surface salinity of the ocean is a key variable in the climate system when studying the global water cycle, ocean–atmosphere exchanges and ocean circulation, all vital components transporting heat, momentum, carbon and nutrients around the world.[80] Cold water is more dense than warm water and salty water is more dense than freshwater. This means the density of ocean water changes as its temperature and salinity changes. These changes in density are the main source of the power that drives the ocean circulation.[80]
Surface ocean salinity measurements taken since the 1950s indicate an intensification of the global water cycle with high saline areas becoming more saline and low saline areas becoming more less saline.[81][82]
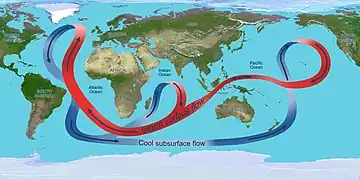
Blue: low salinity Red: high salinity
Ocean acidification
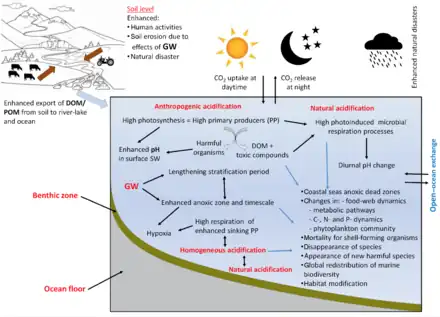
Ocean acidification is the increasing acidification of the oceans, caused mainly by the uptake of carbon dioxide from the atmosphere.[84] The rise in atmospheric carbon dioxide due to the burning of fossil fuels leads to more carbon dioxide dissolving in the ocean. When carbon dioxide dissolves in water it forms hydrogen and carbonate ions. This in turn increases the acidity of the ocean and makes survival increasingly harder for microorganisms, shellfish and other marine organisms that depend on calcium carbonate to form their shells.[85]
Increasing acidity also has potential for other harm to marine organisms, such as depressing metabolic rates and immune responses in some organisms, and causing coral bleaching.[86] Ocean acidification has increased 26% since the beginning of the industrial era.[87] It has been compared to anthropogenic climate change and called the "evil twin of global warming"[88] and "the other CO2 problem".[89]
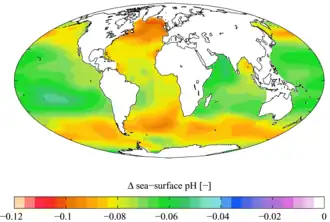
2 from the start of the industrial revolution to the end of the twentieth century
Ocean deoxygenation
Ocean deoxygenation is an additional stressor on marine life. Ocean deoxygenation is the expansion of oxygen minimum zones in the oceans as a consequence of burning fossil fuels. The change has been fairly rapid and poses a threat to fish and other types of marine life, as well as to people who depend on marine life for nutrition or livelihood.[90][91][92][93] Ocean deoxygenation poses implications for ocean productivity, nutrient cycling, carbon cycling, and marine habitats.[94][95]
Ocean warming exacerbates ocean deoxygenation and further stresses marine organisms, limiting nutrient availability by increasing ocean stratification through density and solubility effects while at the same time increasing metabolic demand.[96][97] According to the IPCC 2019 Special Report on the Ocean and Cryosphere in a Changing Climate, the viability of species is being disrupted throughout the ocean food web due to changes in ocean chemistry. As the ocean warms, mixing between water layers decreases, resulting in less oxygen and nutrients being available for marine life.[98]
Polar ice sheets
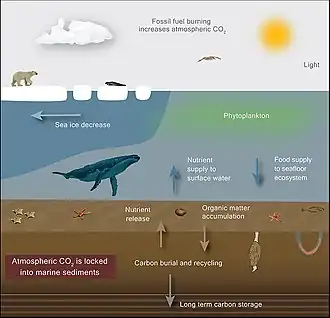
Until recently, ice sheets[100] were viewed as inert components of the carbon cycle and largely disregarded in global models. Research in the past decade has transformed this view, demonstrating the existence of uniquely adapted microbial communities, high rates of biogeochemical/physical weathering in ice sheets and storage and cycling of organic carbon in excess of 100 billion tonnes, as well as nutrients.[101]
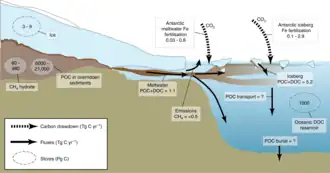
Estimated carbon fluxes are measured in Tg C a−1 (megatonnes of carbon per year) and estimated sizes of carbon stores are measured in Pg C (thousands of megatonnes of carbon). DOC = dissolved organic carbon, POC = particulate organic carbon.[101]
Biogeochemical
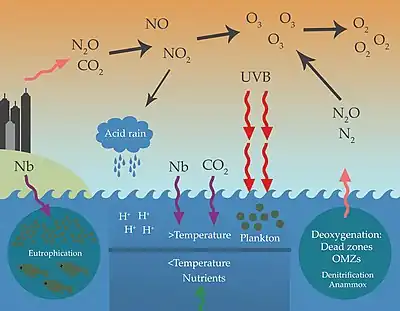
The diagram on the right shows some human impacts on the marine nitrogen cycle. Bioavailable nitrogen (Nb) is introduced into marine ecosystems by runoff or atmospheric deposition, causing eutrophication, the formation of dead zones and the expansion of the oxygen minimum zones (OMZs). The release of nitrogen oxides (N2O, NO) from anthropogenic activities and oxygen-depleted zones causes stratospheric ozone depletion leading to higher UVB exposition, which produces the damage of marine life, acid rain and ocean warming. Ocean warming causes water stratification, deoxygenation, and the formation of dead zones. Dead zones and OMZs are hotspots for anammox and denitrification, causing nitrogen loss (N2 and N2O). Elevated atmospheric carbon dioxide acidifies seawater, decreasing pH-dependent N-cycling processes such as nitrification, and enhancing N2 fixation.[102]
Calcium carbonates
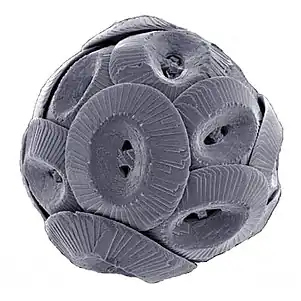
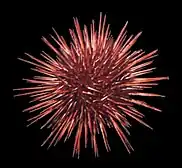
Aragonite is a form of calcium carbonate many marine animals use to build carbonate skeletons and shells. The lower the aragonite saturation level, the more difficult it is for the organisms to build and maintain their skeletons and shells. The map below shows changes in the aragonite saturation level of ocean surface waters between 1880 and 2012.[103]
To pick one example, pteropods are a group of widely distributed swimming sea snails. For pteropods to create shells they require aragonite which is produced through carbonate ions and dissolved calcium. Pteropods are severely affected because increasing acidification levels have steadily decreased the amount of water supersaturated with carbonate which is needed for the aragonite creation.[104]
When the shell of a pteropod was immersed in water with a pH level the ocean is projected to reach by the year 2100, the shell almost completely dissolved within six weeks.[105] Likewise corals,[106] coralline algae,[107] coccolithophores,[108] foraminifera,[109] as well as shellfish generally,[110] all experience reduced calcification or enhanced dissolution as an effect of ocean acidification.
- Decreases in aragonite saturation make it more difficult for marine organisms like pteropods to build calcium shells
- Shells of pteropods dissolve in increasingly acidic conditions caused by increased amounts of atmospheric CO2
.jpg.webp)
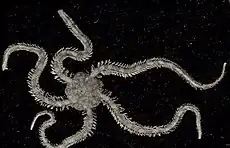
Pteropods and brittle stars together form the base of the Arctic food webs and both are seriously damaged by acidification. Pteropods shells dissolve with increasing acidification and brittle stars lose muscle mass when re-growing appendages.[111] Additionally the brittle star's eggs die within a few days when exposed to expected conditions resulting from Arctic acidification.[112] Acidification threatens to destroy Arctic food webs from the base up. Arctic waters are changing rapidly and are advanced in the process of becoming undersaturated with aragonite.[104] Arctic food webs are considered simple, meaning there are few steps in the food chain from small organisms to larger predators. For example, pteropods are "a key prey item of a number of higher predators – larger plankton, fish, seabirds, whales".[113]
Silicates
The rise in agriculture of the past 400 years has increased the exposure rocks and soils, which has resulted in increased rates of silicate weathering. In turn, the leaching of amorphous silica stocks from soils has also increased, delivering higher concentrations of dissolved silica in rivers.[114] Conversely, increased damming has led to a reduction in silica supply to the ocean due to uptake by freshwater diatoms behind dams. The dominance of non-siliceous phytoplankton due to anthropogenic nitrogen and phosphorus loading and enhanced silica dissolution in warmer waters has the potential to limit silicon ocean sediment export in the future.[114]
In 2019 a group of scientists suggested acidification is reducing diatom silica production in the Southern Ocean.[115][116]
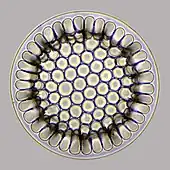
- Concentration of silicic acid in the upper pelagic zone,[117] showing high levels in the Southern Ocean
Carbon


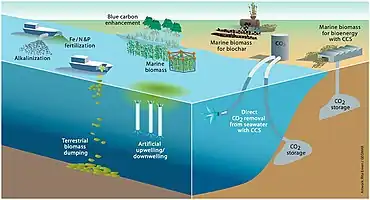
As the technical and political challenges of land-based carbon dioxide removal approaches become more apparent, the oceans may be the new "blue" frontier for carbon drawdown strategies in climate governance.[124] Marine environments are the blue frontier of a strategy for novel carbon sinks in post-Paris climate governance, from nature-based ecosystem management to industrial-scale technological interventions in the Earth system. Marine carbon dioxide removal approaches are diverse [125][126] — although several resemble key terrestrial carbon dioxide removal proposals.[124] Ocean alkalinisation (adding silicate mineral such as olivine to coastal seawater, to increase CO2 uptake through chemical reactions) is enhanced weathering, blue carbon (enhancing natural biological CO2 drawdown from coastal vegetation) is marine reforestation, and cultivation of marine biomass (i.e., seaweed) for coupling with consequent carbon capture and storage is the marine variant of bioenergy and carbon capture and storage. Wetlands, coasts, and the open ocean are being conceived of and developed as managed carbon removal-and-storage sites, with practices expanded from the use of soils and forests.[124]
Effect of multiple stressors

If more than one stressor is present the effects can be amplified.[129][130] For example, the combination of ocean acidification and an elevation of ocean temperature can have a compounded effect on marine life far exceeding the individual harmful impact of either.[131][132][133]
While the full implications of elevated CO2 on marine ecosystems are still being documented, there is a substantial body of research showing that a combination of ocean acidification and elevated ocean temperature, driven mainly by CO2 and other greenhouse gas emissions, have a compounded effect on marine life and the ocean environment. This effect far exceeds the individual harmful impact of either.[131][134][133] In addition, ocean warming exacerbates ocean deoxygenation, which is an additional stressor on marine organisms, by increasing ocean stratification, through density and solubility effects, thus limiting nutrients,[135][136] while at the same time increasing metabolic demand.
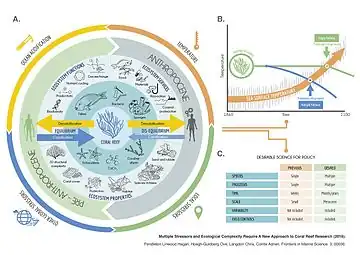
The direction and magnitude of the effects of ocean acidification, warming and deoxygenation on the ocean has been quantified by meta-analyses,[132][138][139] and has been further tested by mesocosm studies. The mesocosm studies simulated the interaction of these stressors and found a catastrophic effect on the marine food web, namely, that the increases in consumption from thermal stress more than negates any primary producer to herbivore increase from more available carbon dioxide.[140][141]
Drivers of change

Changes in marine ecosystem dynamics are influenced by socioeconomic activities (for example, fishing, pollution) and human-induced biophysical change (for example, temperature, ocean acidification) and can interact and severely impact marine ecosystem dynamics and the ecosystem services they generate to society. Understanding these direct—or proximate—interactions is an important step towards sustainable use of marine ecosystems. However, proximate interactions are embedded in a much broader socioeconomic context where, for example, economy through trade and finance, human migration and technological advances, operate and interact at a global scale, influencing proximate relationships.[142]
Shifting baselines
"Application of the physical and biological sciences has made today arguably the best of times: we live longer and healthier lives, food production has doubled in the past 35 years and energy subsidies have substituted for human labour, washing away hierarchies of servitude. But the unintended consequences of these well-intentioned actions — climate change, biodiversity loss, inadequate water supplies, and much else — could well make tomorrow the worst of times."
– Robert May 2006 [143]
Shifting baselines arise in research on marine ecosystems because changes must be measured against some previous reference point (baseline), which in turn may represent significant changes from an even earlier state of the ecosystem.[144] For example, radically depleted fisheries have been evaluated by researchers who used the state of the fishery at the start of their careers as the baseline, rather than the fishery in its unexploited or untouched state. Areas that swarmed with a particular species hundreds of years ago may have experienced long-term decline, but it is the level a few decades previously that is used as the reference point for current populations. In this way large declines in ecosystems or species over long periods of time were, and are, masked. There is a loss of perception of change that occurs when each generation redefines what is natural or untouched.[144]
See also
- Anthropocene
- Climate change and ecosystems
- Deep-sea exploration
- Effects of climate change on oceans
- Effects of climate change on terrestrial animals
- Effects of global warming on marine mammals
- Garbage patch
- Marine debris
- Oceanic carbon cycle
- Offshore construction
- Ocean exploration
- Special Report on the Ocean and Cryosphere in a Changing Climate
- Sustainable Development Goal 14
- Threatened rays
- Threatened sharks
- World Oceans Day
References
- 1 2 Halpern, B.S., Frazier, M., Afflerbach, J. et al. (2019) "Recent pace of change in human impact on the world’s ocean." Scientific Reports, 9: 11609. doi:10.1038/s41598-019-47201-9
- ↑ Halpern, B.S., Walbridge, S., Selkoe, K.A., Kappel, C.V., Micheli, F., D'agrosa, C., Bruno, J.F., Casey, K.S., Ebert, C., Fox, H.E. and Fujita, R. (2008) "A global map of human impact on marine ecosystems". Science, 319(5865): 948–952. doi:10.1126/science.1149345
- ↑ Human impacts on marine ecosystems GEOMAR Helmholtz Centre for Ocean Research. Retrieved 22 October 2019.
- ↑ Special Report on the Ocean and Cryosphere in a Changing Climate (SROCC). IPCC (Report). 25 September 2019. p. 2. Retrieved 25 March 2020.
- ↑ Jones, K.R., Klein, C.J., Halpern, B.S., Venter, O., Grantham, H., Kuempel, C.D., Shumway, N., Friedlander, A.M., Possingham, H.P. and Watson, J.E. (2018) "The location and protection status of Earth’s diminishing marine wilderness". Current Biology, 28(15): 2506–2512. doi:10.1016/j.cub.2018.06.010
- ↑ fao.org. "SOFIA 2018 - State of Fisheries and Aquaculture in the world 2018". www.fao.org. Retrieved 9 November 2018.
- ↑ World Wildlife Fund. "Fishing problems: Illegal fishing" Archived 2008-04-11 at the Wayback Machine
- ↑ Pauly, Daniel and Watson, Reg (2009) "Spatial Dynamics of Marine Fisheries" Archived 2012-06-11 at the Wayback Machine In: Simon A. Levin (ed.) The Princeton Guide to Ecology. Pages 501–509.
- ↑ Pauly, Daniel. Fisheries on the brink (YouTube video). Retrieved 1 May 2012.
- 1 2 Waycott, M., Duarte, C.M., Carruthers, T.J., Orth, R.J., Dennison, W.C., Olyarnik, S., Calladine, A., Fourqurean, J.W., Heck, K.L., Hughes, A.R. and Kendrick, G.A. (2009) "Accelerating loss of seagrasses across the globe threatens coastal ecosystems". Proceedings of the national academy of sciences, 106(30): 12377–12381. doi:10.1073/pnas.0905620106
- ↑ Wilkinson, Clive (2008) Status of Coral Reefs of the World: Executive Summary. Global Coral Reef Monitoring Network.
- ↑ Zainal Abidin, Siti Zulaiha; Mohamed, Badaruddin (2014). Othuman Mydin, M.A.; Marzuki, A. (eds.). "A Review of SCUBA Diving Impacts and Implication for Coral Reefs Conservation and Tourism Management". SHS Web of Conferences. 12: 01093. doi:10.1051/shsconf/20141201093. ISSN 2261-2424.
- ↑ Vanwonterghem, I. and Webster, N.S. (2020) "Coral reef microorganisms in a changing climate". Iscience, 23(4). doi:10.1016/j.isci.2020.100972.
- ↑ "2010a. ""World Atlas of Mangroves" Highlights the Importance of and Threats to Mangroves: Mangroves among World's Most Valuable Ecosystems." Press release. Arlington, Virginia". The Nature Conservancy. Archived from the original on 17 July 2010. Retrieved 25 January 2014.
- ↑ Sala, E., C.F. Bourdouresque and M. Harmelin-Vivien. 1998. Fishing, trophic cascades, and the structure of algal assemblages: evaluation of an old but untested paradigm. Oikos 82: 425-439.
- ↑ Joan G. Ehrenfeld (2010), "Ecosystem Consequences of Biological Invasions", Annual Review of Ecology, Evolution, and Systematics, 41: 59–80, doi:10.1146/annurev-ecolsys-102209-144650
- ↑ Molnar, Jennifer L; Gamboa, Rebecca L; Revenga, Carmen; Spalding, Mark D (2008). "Assessing the global threat of invasive species to marine biodiversity". Frontiers in Ecology and the Environment. 6 (9): 485–492. doi:10.1890/070064. ISSN 1540-9295.
- ↑ Aquatic invasive species. A Guide to Least-Wanted Aquatic Organisms of the Pacific Northwest Archived 25 July 2008 at the Wayback Machine. 2001. University of Washington
- ↑ Meinesz, A. (2003) Deep Sea Invasion: The Impact of Invasive Species PBS: NOVA. Retrieved 26 November 2009
- ↑ Pimentel, D.; Zuniga, R.; Morrison, D. (2005). "Update on the environmental and economic costs associated with alien-invasive species in the United States". Ecological Economics. 52 (3): 273–288. doi:10.1016/j.ecolecon.2004.10.002.
- ↑ Charles Sheppard, ed. (2019). World seas : an Environmental Evaluation. Vol. III, Ecological Issues and Environmental Impacts (Second ed.). London, United Kingdom. ISBN 978-0-12-805204-4. OCLC 1052566532.
- ↑ Duce, Robert, Galloway, J. and Liss, P. (2009). "The Impacts of Atmospheric Deposition to the Ocean on Marine Ecosystems and Climate WMO Bulletin Vol 58 (1)". Retrieved 22 September 2020.
- ↑ US Department of Commerce, National Oceanic and Atmospheric Administration. "What is the biggest source of pollution in the ocean?". oceanservice.noaa.gov. Retrieved 22 November 2015.
- ↑ Breitburg, Denise; Levin, Lisa A.; Oschlies, Andreas; Grégoire, Marilaure; Chavez, Francisco P.; Conley, Daniel J.; Garçon, Véronique; Gilbert, Denis; Gutiérrez, Dimitri; Isensee, Kirsten; Jacinto, Gil S. (5 January 2018). "Declining oxygen in the global ocean and coastal waters". Science. 359 (6371): eaam7240. Bibcode:2018Sci...359M7240B. doi:10.1126/science.aam7240. ISSN 0036-8075. PMID 29301986.
- ↑ US Department of Commerce, National Oceanic and Atmospheric Administration. "What is a dead zone?". oceanservice.noaa.gov. Retrieved 30 April 2021.
- ↑ "Nutrient Pollution". Ocean Health Index. Retrieved 30 April 2021.
- ↑ Boyce, D.G. and Worm, B. (2015) "Patterns and ecological implications of historical marine phytoplankton change". Marine Ecology Progress Series, 534:251–272. doi:10.3354/meps11411
- ↑ "Marine plastic pollution". IUCN. 25 May 2018. Retrieved 31 January 2022.
- 1 2 US EPA, OW (6 November 2020). "Plastic Pollution". US EPA. Retrieved 30 April 2021.
- ↑ "Discover the plastic islands that pollute our oceans". Iberdrola. Retrieved 30 April 2021.
- ↑ "Marine plastics". IUCN. 25 May 2018. Retrieved 30 April 2021.
- ↑ "We Depend on Plastic. Now We're Drowning in It". Magazine. 16 May 2018. Retrieved 30 April 2021.
- ↑ "Marine Microplastics". Woods Hole Oceanographic Institution. Retrieved 30 April 2021.
- ↑ "Great Pacific Garbage Patch". Marine Debris Division – Office of Response and Restoration. NOAA. 11 July 2013. Archived from the original on 17 April 2014. Retrieved 7 December 2019.
- ↑ Eriksen, M., Lebreton, L.C., Carson, H.S., Thiel, M., Moore, C.J., Borerro, J.C., Galgani, F., Ryan, P.G. and Reisser, J. (2014) "Plastic pollution in the world's oceans: more than 5 trillion plastic pieces weighing over 250,000 tons afloat at sea". PLOS ONE, 9 (12): e111913. doi:10.1371/journal.pone.0111913.g002
- ↑ Urbanek, A.K., Rymowicz, W. and Mirończuk, A.M. (2018) "Degradation of plastics and plastic-degrading bacteria in cold marine habitats". Applied microbiology and biotechnology, 102(18): 7669–7678. doi:10.1007/s00253-018-9195-y.
- ↑ "Underwater Noise Pollution Is Disrupting Ocean Life—But We Can Fix It". Time. Retrieved 30 April 2021.
- ↑ "The Ocean is Too Loud for Marine Life". Discovery. Retrieved 30 April 2021.
- ↑ Society, National Geographic (16 July 2019). "Noise Pollution". National Geographic Society. Retrieved 30 April 2021.
- ↑ "David Attenborough calls for ban on 'devastating' deep sea mining". the Guardian. 12 March 2020. Retrieved 11 September 2021.
- ↑ Halfar, Jochen; Fujita, Rodney M. (18 May 2007). "Danger of Deep-Sea Mining". Science. 316 (5827): 987. doi:10.1126/science.1138289. PMID 17510349. S2CID 128645876.
- 1 2 Charles Sheppard, ed. (2019). World seas : an Environmental Evaluation. Vol. III, Ecological Issues and Environmental Impacts (Second ed.). London, United Kingdom. ISBN 978-0-12-805204-4. OCLC 1052566532.
- 1 2 Cavicchioli, R., Ripple, W.J., Timmis, K.N., Azam, F., Bakken, L.R., Baylis, M., Behrenfeld, M.J., Boetius, A., Boyd, P.W., Classen, A.T. and Crowther, T.W. (2019) "Scientists’ warning to humanity: microorganisms and climate change". Nature Reviews Microbiology, 17: 569–586. doi:10.1038/s41579-019-0222-5
- ↑ Käse, Laura; Geuer, Jana K. (2018). "Phytoplankton Responses to Marine Climate Change – an Introduction". YOUMARES 8 – Oceans Across Boundaries: Learning from each other. pp. 55–71. doi:10.1007/978-3-319-93284-2_5. ISBN 978-3-319-93283-5.
Modified text was copied from this source, which is available under a Creative Commons Attribution 4.0 International License.
- 1 2 IPCC (2007) Ocean Heat Content Fourth Assessment Report.
- 1 2 Nuccitelli et al 2012 Total Heat Content Skeptical Science. Accessed 30 December 2019.
- ↑ Hansen, J., Fung, I., Lacis, A., Rind, D., Lebedeff, S., Ruedy, R., Russell, G. and Stone, P. (1988) "Global climate changes as forecast by Goddard Institute for Space Studies three‐dimensional model". Journal of geophysical research: Atmospheres, 93(D8): 9341–9364. doi:10.1029/JD093iD08p09341
- ↑ Data source: NOAA and Rutgers University (2016) OceanAdapt
- ↑ Pinsky, M.L., Worm, B., Fogarty, M.J., Sarmiento, J.L. and Levin, S.A. (2013) "Marine taxa track local climate velocities". Science, 341(6151): 1239–1242. doi:10.1126/science.1239352
- ↑ Nuccitelli, D., Way, R., Painting, R., Church, J. and Cook, J. (2012) "Comment on 'Ocean heat content and Earthʼs radiation imbalance. II. Relation to climate shifts'". Physics Letters A, 376(45): 3466–3468. doi:10.1016/j.physleta.2012.10.010
- 1 2 Roxy, M.K. (2016). "A reduction in marine primary productivity driven by rapid warming over the tropical Indian Ocean" (PDF). Geophysical Research Letters. 43 (2): 826–833. Bibcode:2016GeoRL..43..826R. doi:10.1002/2015GL066979.
- 1 2 3 4 Climate Change Could Threaten Many Antarctic Marine Species Pew, 25 October 2019.
- 1 2 3 4 Rogers, A.D., Frinault, B.A.V., Barnes, D.K.A., Bindoff, N.L., Downie, R., Ducklow, H.W., Friedlaender, A.S., Hart, T., Hill, S.L., Hofmann, E.E. and Linse, K. (2019) "Antarctic futures: an assessment of climate-driven changes in ecosystem structure, function, and service provisioning in the Southern Ocean". Annual review of marine science, 12: 87–120. doi:10.1146/annurev-marine-010419-011028
- ↑ Australian Bureau of Meteorology - The Southern Annular Mode. Accessed 25/10/2013. http://www.bom.gov.au/climate/enso/history/ln-2010-12/SAM-what.shtml
- ↑ Thompson, David W. J.; Solomon, Susan; Kushner, Paul J.; England, Matthew H.; Grise, Kevin M.; Karoly, David J. (23 October 2011). "Signatures of the Antarctic ozone hole in Southern Hemisphere surface climate change". Nature Geoscience. 4 (11): 741–749. Bibcode:2011NatGe...4..741T. doi:10.1038/ngeo1296. ISSN 1752-0894.
- ↑ Hayakawa, Hideaki; Shibuya, Kazuo; Aoyama, Yuichi; Nogi, Yoshifumi; Doi, Koichiro (2012). "Ocean bottom pressure variability in the Antarctic Divergence Zone off Lützow-Holm Bay, East Antarctica". Deep Sea Research Part I: Oceanographic Research Papers. 60: 22–31. Bibcode:2012DSRI...60...22H. doi:10.1016/j.dsr.2011.09.005. ISSN 0967-0637.
- ↑ Spence, Paul; Griffies, Stephen M.; England, Matthew H.; Hogg, Andrew McC.; Saenko, Oleg A.; Jourdain, Nicolas C. (12 July 2014). "Rapid subsurface warming and circulation changes of Antarctic coastal waters by poleward shifting winds" (PDF). Geophysical Research Letters. 41 (13): 4601–4610. Bibcode:2014GeoRL..41.4601S. doi:10.1002/2014gl060613. hdl:1885/56321. ISSN 0094-8276.
- ↑ Greene, Chad A.; Blankenship, Donald D.; Gwyther, David E.; Silvano, Alessandro; Wijk, Esmee van (1 November 2017). "Wind causes Totten Ice Shelf melt and acceleration". Science Advances. 3 (11): e1701681. Bibcode:2017SciA....3E1681G. doi:10.1126/sciadv.1701681. ISSN 2375-2548. PMC 5665591. PMID 29109976.
- ↑ Anderson, R. F.; Ali, S.; Bradtmiller, L. I.; Nielsen, S. H. H.; Fleisher, M. Q.; Anderson, B. E.; Burckle, L. H. (13 March 2009). "Wind-Driven Upwelling in the Southern Ocean and the Deglacial Rise in Atmospheric CO2". Science. 323 (5920): 1443–1448. Bibcode:2009Sci...323.1443A. doi:10.1126/science.1167441. ISSN 0036-8075. PMID 19286547. S2CID 206517043.
- ↑ "1000-year Southern Annular Mode Reconstruction". NOAA: National Climatic Data Center. Retrieved 5 January 2020.
- ↑ Abram, Nerilie (11 May 2014). "Evolution of the Southern Annular Mode during the past millennium". Nature. Retrieved 13 September 2014.
- ↑ Mario Vacchi; Philippe Koubbi; Laura Ghigliotti; Eva Pisano (2012). "Sea-ice interactions with polar fish: focus on the Antarctic silverfish life history". In Guido di Prisco; Cinzia Verde (eds.). The Impacts of Global Change on Biodiversity. Adaptation and Evolution in Marine Environments. Vol. 1. Springer Science & Business Media. pp. 51–73. doi:10.1007/978-3-642-27352-0_4. ISBN 9783642273513.
- ↑ Chapter 5: Changing Ocean, Marine Ecosystems, and Dependent Communities (PDF). IPCC (Report). Special Report on the Ocean and Cryosphere in a Changing Climate (SROCC). 25 September 2019. pp. 5–6. Retrieved 25 March 2020.
- ↑ "'We're All in Big Trouble': Climate Panel Sees a Dire Future". The New York Times via the Associated Press. 25 September 2019. Retrieved 25 March 2020.
- ↑ Climate change in deep oceans could be seven times faster by middle of century, report says The Guardian, 25 May 2020.
- ↑ Brito-Morales, I., Schoeman, D.S., Molinos, J.G., Burrows, M.T., Klein, C.J., Arafeh-Dalmau, N., Kaschner, K., Garilao, C., Kesner-Reyes, K. and Richardson, A.J. (2020) "Climate velocity reveals increasing exposure of deep-ocean biodiversity to future warming". Nature Climate Change, pp.1-6. doi:10.5281/zenodo.3596584.
- ↑ Lindsey, Rebecca (2019) Climate Change: Global Sea Level NOAA Climate, 19 November 2019.
- ↑ "Sea level rise poses a major threat to coastal ecosystems and the biota they support". birdlife.org. Birdlife International. 2015.
- ↑ Pontee, Nigel (November 2013). "Defining coastal squeeze: A discussion". Ocean & Coastal Management. 84: 204–207. doi:10.1016/j.ocecoaman.2013.07.010.
- ↑ Krauss, Ken W.; McKee, Karen L.; Lovelock, Catherine E.; Cahoon, Donald R.; Saintilan, Neil; Reef, Ruth; Chen, Luzhen (April 2014). "How mangrove forests adjust to rising sea level". New Phytologist. 202 (1): 19–34. doi:10.1111/nph.12605. PMID 24251960.
- ↑ CSIRO 2015 update to data originally published in: Church, J.A., and N.J. White (2011) "Sea-level rise from the late 19th to the early 21st century". Surv. Geophys., 32: 585–602.
- ↑ NOAA Laboratory for Satellite Altimetry (2016) Global sea level time series. Accessed: June 2016.
- ↑ Wong, Poh Poh; Losado, I.J.; Gattuso, J.-P.; Hinkel, Jochen (2014). "Coastal Systems and Low-Lying Areas" (PDF). Climate Change 2014: Impacts, Adaptation, and Vulnerability. New York: Cambridge University Press.
- ↑ Crosby, Sarah C.; Sax, Dov F.; Palmer, Megan E.; Booth, Harriet S.; Deegan, Linda A.; Bertness, Mark D.; Leslie, Heather M. (November 2016). "Salt marsh persistence is threatened by predicted sea-level rise". Estuarine, Coastal and Shelf Science. 181: 93–99. Bibcode:2016ECSS..181...93C. doi:10.1016/j.ecss.2016.08.018.
- ↑ Spalding M.; McIvor A.; Tonneijck F.H.; Tol S.; van Eijk P. (2014). "Mangroves for coastal defence. Guidelines for coastal managers & policy makers" (PDF). Wetlands International and The Nature Conservancy.
- ↑ Weston, Nathaniel B. (16 July 2013). "Declining Sediments and Rising Seas: an Unfortunate Convergence for Tidal Wetlands". Estuaries and Coasts. 37 (1): 1–23. doi:10.1007/s12237-013-9654-8. S2CID 128615335.
- ↑ "Sea Level Rise". National Geographic. 13 January 2017.
- ↑ Smith, Lauren (15 June 2016). "Extinct: Bramble Cay melomys". Australian Geographic. Retrieved 17 June 2016.
- ↑ Hannam, Peter (19 February 2019). "'Our little brown rat': first climate change-caused mammal extinction". The Sydney Morning Herald. Retrieved 25 June 2019.
- 1 2 New maps of salinity reveal the impact of climate variability on oceans European Space Agency, 2 December 2019, PHYS.ORG.
- ↑ Gillis, Justin (26 April 2012). "Study Indicates a Greater Threat of Extreme Weather". The New York Times. Archived from the original on 26 April 2012. Retrieved 27 April 2012.
- ↑ Vinas, Maria-Jose (6 June 2013). "NASA's Aquarius Sees Salty Shifts". NASA. Archived from the original on 16 May 2017. Retrieved 15 January 2018.
- ↑ Mostofa, K.M., Liu, C.Q., Zhai, W., Minella, M., Vione, D.V., Gao, K., Minakata, D., Arakaki, T., Yoshioka, T., Hayakawa, K. and Konohira, E. (2016) "Reviews and Syntheses: Ocean acidification and its potential impacts on marine ecosystems". Biogeosciences, 13: 1767–1786. doi:10.5194/bg-13-1767-2016.
Modified text was copied from this source, which is available under a Creative Commons Attribution 3.0 International License.
- ↑ Caldeira, K.; Wickett, M. E. (2003). "Anthropogenic carbon and ocean pH". Nature. 425 (6956): 365. Bibcode:2001AGUFMOS11C0385C. doi:10.1038/425365a. PMID 14508477. S2CID 4417880.
- ↑ Trujillo AP and Thurman HV (2009) Essentials of Oceanography, 9th edition, page 151, Pearson Education International: ISBN 9780138150709
- ↑ Anthony, KRN; et al. (2008). "Ocean acidification causes bleaching and productivity loss in coral reef builders". Proceedings of the National Academy of Sciences. 105 (45): 17442–17446. Bibcode:2008PNAS..10517442A. doi:10.1073/pnas.0804478105. PMC 2580748. PMID 18988740.
- ↑ IPCC (2019) Summary for Policymakers. In: IPCC Special Report on the Ocean and Cryosphere in a Changing Climate, Chapter 1, page 14. [H.O. Pörtner, D.C. Roberts, V. Masson-Delmotte, P. Zhai, M. Tignor, E. Poloczanska, K. Mintenbeck, M. Nicolai, A. Okem, J. Petzold, B. Rama, N. Weyer (eds.)]. Final draft: 24 September 2019.
- ↑ "Ocean Acidification Is Climate Change's 'Equally Evil Twin,' NOAA Chief Says". Huffington Post. 9 July 2012. Archived from the original on 12 July 2012. Retrieved 9 July 2012.
- ↑ Doney, S.C.; Fabry, V.J.; Feely, R.A.; Kleypas, J.A. (2009). "Ocean Acidification: The Other CO2 Problem" (PDF). Annual Review of Marine Science. 1: 169–192. Bibcode:2009ARMS....1..169D. doi:10.1146/annurev.marine.010908.163834. PMID 21141034. S2CID 402398. Archived from the original (PDF) on 22 February 2019.
- ↑ Oceans suffocating as huge dead zones quadruple since 1950, scientists warn The Guardian, 2018
- ↑ Ocean's Oxygen Starts Running Low
- ↑ Finding forced trends in oceanic oxygen
- ↑ How global warming is causing ocean oxygen levels to fall
- ↑ Harvey, Fiona (7 December 2019). "Oceans losing oxygen at unprecedented rate, experts warn". The Guardian. ISSN 0261-3077. Retrieved 7 December 2019.
- ↑ Laffoley, D. and Baxter, J. M. (eds.) (2019) Ocean deoxygenation : everyone’s problem, IUCN Report.
- ↑ Bednaršek, N., Harvey, C.J., Kaplan, I.C., Feely, R.A. and Možina, J. (2016) "Pteropods on the edge: Cumulative effects of ocean acidification, warming, and deoxygenation". Progress in Oceanography, 145: 1–24. doi:10.1016/j.pocean.2016.04.002
- ↑ Keeling, Ralph F., and Hernan E. Garcia (2002) "The change in oceanic O2 inventory associated with recent global warming." Proceedings of the National Academy of Sciences, 99(12): 7848–7853. doi:10.1073/pnas.122154899
- ↑ "Press Release" (PDF). IPCC (Press release). Special Report on the Ocean and Cryosphere in a Changing Climate (SROCC). 25 September 2019. p. 3. Retrieved 25 March 2020.
- ↑ Faust, Johan C.; März, Christian; Henley, Sian F. (2019). "The Carbon Story of a Melting Arctic". Frontiers for Young Minds. 7. doi:10.3389/frym.2019.00136. S2CID 208531858.
Modified text was copied from this source, which is available under a Creative Commons Attribution 4.0 International License.
- ↑ "Quick Facts on Ice Sheets | National Snow and Ice Data Center". nsidc.org. Retrieved 14 September 2021.
- 1 2 Wadham, J.L., Hawkings, J.R., Tarasov, L., Gregoire, L.J., Spencer, R.G.M., Gutjahr, M., Ridgwell, A. and Kohfeld, K.E. (2019) "Ice sheets matter for the global carbon cycle". Nature communications, 10(1): 1–17. doi:10.1038/s41467-019-11394-4.
Modified text was copied from this source, which is available under a Creative Commons Attribution 4.0 International License.
- 1 2 Silvia Pajares; Ramiro Ramos (29 November 2019). "Processes and Microorganisms Involved in the Marine Nitrogen Cycle: Knowledge and Gaps". Frontiers in Marine Science. 6. doi:10.3389/fmars.2019.00739.
Modified text was copied from this source, which is available under a Creative Commons Attribution 4.0 International License.
- ↑ Woods Hole Oceanographic Institution (August 2016). "Changes in Aragonite Saturation of the World's Oceans, 1880–2015".
Feely, R.A.; Doney, S.C.; Cooley, S.R. (2009). "Ocean acidification: Present conditions and future changes in a high-CO2 world" (PDF). Oceanography. 22 (4): 36–47. doi:10.5670/oceanog.2009.95. hdl:1912/3180 – via Woods Hole Open Access Server.
"Climate Change Indicators in the United States, 2012, 2nd ed: Ocean Acidity: Figure 2. Changes in Aragonite Saturation of the World's Oceans, 1880-2012". US Environmental Protection Agency (EPA). - 1 2 Lischka, S.; Büdenbender J.; Boxhammer T.; Riebesell U. (15 April 2011). "Impact of ocean acidification and elevated temperatures on early juveniles of the polar shelled pteropod Limacina helicina : mortality, shell degradation, and shell growth" (PDF). Report. Biogeosciences. pp. 919–932. Retrieved 14 November 2013.
- ↑ Bednaršek, N.; Feely, R. A.; Reum, J. C. P.; Peterson, B.; Menkel, J.; Alin, S. R.; Hales, B. (2014). "Limacina helicina shell dissolution as an indicator of declining habitat suitability owing to ocean acidification in the California Current Ecosystem". Proc. R. Soc. B. 281 (1785): 20140123. doi:10.1098/rspb.2014.0123. ISSN 0962-8452. PMC 4024287. PMID 24789895.
- ↑ D'Olivo, Juan P.; Ellwood, George; DeCarlo, Thomas M.; McCulloch, Malcolm T. (15 November 2019). "Deconvolving the long-term impacts of ocean acidification and warming on coral biomineralisation". Earth and Planetary Science Letters. 526: 115785. Bibcode:2019E&PSL.52615785D. doi:10.1016/j.epsl.2019.115785. ISSN 0012-821X.
- ↑ Kuffner, I. B.; Andersson, A. J.; Jokiel, P. L.; Rodgers, K. S.; Mackenzie, F. T. (2007). "Decreased abundance of crustose coralline algae due to ocean acidification". Nature Geoscience. 1 (2): 114–117. Bibcode:2008NatGe...1..114K. doi:10.1038/ngeo100. S2CID 3456369.
- ↑ Delille, B.; Harlay, J.; Zondervan, I.; Jacquet, S.; Chou, L.; Wollast, R.; Bellerby, R.G.J.; Frankignoulle, M.; Borges, A.V.; Riebesell, U.; Gattuso, J.-P. (2005). "Response of primary production and calcification to changes of pCO2 during experimental blooms of the coccolithophorid Emiliania huxleyi". Global Biogeochemical Cycles. 19 (2): GB2023. Bibcode:2005GBioC..19.2023D. doi:10.1029/2004GB002318.
- ↑ Phillips, Graham; Chris Branagan (2007). "Ocean Acidification – The BIG global warming story". ABC TV Science: Catalyst. Australian Broadcasting Corporation. Retrieved 18 September 2007.
- ↑ Gazeau, F.; Quiblier, C.; Jansen, J. M.; Gattuso, J.-P.; Middelburg, J. J.; Heip, C. H. R. (2007). "Impact of elevated CO
2 on shellfish calcification". Geophysical Research Letters. 34 (7): L07603. Bibcode:2007GeoRL..3407603G. doi:10.1029/2006GL028554. hdl:20.500.11755/a8941c6a-6d0b-43d5-ba0d-157a7aa05668. - ↑ "Effects of Ocean Acidification on Marine Species & Ecosystems". Report. OCEANA. Retrieved 13 October 2013.
- ↑ "Comprehensive study of Arctic Ocean acidification". Study. CICERO. Retrieved 14 November 2013.
- ↑ "Antarctic marine wildlife is under threat, study finds". BBC Nature. Retrieved 13 October 2013.
- 1 2 Gaillardet, J.; Dupré, B.; Louvat, P.; Allègre, C.J. (July 1999). "Global silicate weathering and CO2 consumption rates deduced from the chemistry of large rivers". Chemical Geology. 159 (1–4): 3–30. Bibcode:1999ChGeo.159....3G. doi:10.1016/s0009-2541(99)00031-5. ISSN 0009-2541.
- ↑ New threat from ocean acidification emerges in the Southern Ocean, Phys.org, 26 August 2019.
- ↑ Petrou, K., Baker, K.G., Nielsen, D.A. et al. (2019) "Acidification diminishes diatom silica production in the Southern Ocean". Nature: Climate Change, 9: 781–786. doi:10.1038/s41558-019-0557-y
- ↑ Information, US Department of Commerce, NOAA National Centers for Environmental. "World Ocean Atlas 2009". www.nodc.noaa.gov. Retrieved 17 April 2018.
- ↑ Tréguer, Paul; Nelson, David M.; Bennekom, Aleido J. Van; DeMaster, David J.; Leynaert, Aude; Quéguiner, Bernard (21 April 1995). "The Silica Balance in the World Ocean: A Reestimate". Science. 268 (5209): 375–379. Bibcode:1995Sci...268..375T. doi:10.1126/science.268.5209.375. ISSN 0036-8075. PMID 17746543. S2CID 5672525.
- ↑ Ciais, P., Sabine, C., Govindasamy, B., Bopp, L., Brovkin, V., Canadell, J., Chhabra, A., DeFries, R., Galloway, J., Heimann, M., Jones, C., Le Quéré, C., Myneni, R., Piao, S., and Thornton, P.: Chapter 6: Carbon and Other Biogeochemical Cycles, in: Climate Change 2013 The Physical Science Basis, edited by: Stocker, T., Qin, D., and Platner, G.-K., Cambridge University Press, Cambridge, 2013.
- ↑ Price, J. T. and Warren, R (2016) Review of the Potential of “Blue Carbon” Activities to Reduce Emissions.
- ↑ Friedlingstein, P., Jones, M., O'Sullivan, M., Andrew, R., Hauck, J., Peters, G., Peters, W., Pongratz, J., Sitch, S., Le Quéré, C. and 66 others (2019) "Global carbon budget 2019". Earth System Science Data, 11(4): 1783–1838. doi:10.5194/essd-11-1783-2019.
Modified text was copied from this source, which is available under a Creative Commons Attribution 4.0 International License.
- ↑ Cardini, U., Bednarz, V.N., Foster, R.A. and Wild, C. (2014) "Benthic N2 fixation in coral reefs and the potential effects of human‐induced environmental change". Ecology and evolution, 4(9): 1706–1727. doi:10.1002/ece3.1050
Modified text was copied from this source, which is available under a Creative Commons Attribution 4.0 International License.
- ↑ Adapted from: Gruber, N., and J. N. Galloway (2008) "An Earth‐system perspective of the global nitrogen cycle". Nature, 451:293–296. doi:10.1038/nature06592.
- 1 2 3 4 Boettcher, Miranda; Brent, Kerryn; Buck, Holly Jean; Low, Sean; McLaren, Duncan; Mengis, Nadine (2021). "Navigating Potential Hype and Opportunity in Governing Marine Carbon Removal". Frontiers in Climate. 3. doi:10.3389/fclim.2021.664456.
Modified text was copied from this source, which is available under a Creative Commons Attribution 4.0 International License.
- ↑ Royal Society/Royal Academy of Engineering (2018). Greenhouse Gas Removal. ISBN 978-1-78252-349-9
- ↑ GESAMP (2019). "High level review of a wide range of proposed marine geoengineering techniques", in Rep. Stud. GESAMP. 98, (IMO/FAO/UNESCO-IOC/UNIDO/WMO/IAEA/UN/UN Environment/ UNDP/ISA Joint Group of Experts on the Scientific Aspects of Marine Environmental Protection). Eds: P. W. Boyd and C. M. G. Vivian, International Maritime Organization, London.
- ↑ Chan, F., Barth, J.A., Kroeker, K.J., Lubchenco, J. and Menge, B.A. (2019) "The dynamics and impact of ocean acidification and hypoxia". Oceanography, 32(3): 62–71. doi:10.5670/oceanog.2019.312.
Modified text was copied from this source, which is available under a Creative Commons Attribution 4.0 International License.
- ↑ Gewin, V. (2010) "Oceanography: Dead in the water". Nature, 466(7308): 812. doi:10.1038/466812a.
- ↑ Breitburg, D.L. and Riedel, G.F. (2005) "Multiple stressors in marine systems". In: M. E. Soulé, Marine conservation biology: the science of maintaining the sea's biodiversity, Island Press, pages 167–182. ISBN 9781597267717
- ↑ Bopp, L., Resplandy, L., Orr, J.C., Doney, S.C., Dunne, J.P., Gehlen, M., Halloran, P., Heinze, C., Ilyina, T., Seferian, R. and Tjiputra, J. (2013) "Multiple stressors of ocean ecosystems in the 21st century: projections with CMIP5 models". Biogeosciences, 10: 6225–6245. doi:10.5194/bg-10-6225-2013
- 1 2 Kroeker, et al. (June 2013) "Impacts of ocean acidification on marine organisms: quantifying sensitivities and interaction with warming." Glob Chang Biol. 19(6): 1884–1896
- 1 2 Harvey B.P., Gwynn‐Jones D. and Moore P.J. (2013) "Meta‐analysis reveals complex marine biological responses to the interactive effects of ocean acidification and warming". Ecology and evolution, 3(4): 1016–1030. doi:10.1002/ece3.516
- 1 2 Nagelkerken Global alteration of ocean ecosystem functioning due to increasing human CO2 emissions, PNAS vol. 112 no. 43, 2015
- ↑ Harvey, et al. (April 2013) "Meta-analysis reveals complex marine biological responses to the interactive effects of ocean acidification and warming." Ecol Evol. 3(4): 1016–1030
- ↑ Bednaršek, N.; Harvey, C.J.; Kaplan, I.C.; Feely, R.A.; Možina, J. (2016). "Pteropods on the edge: Cumulative effects of ocean acidification, warming, and deoxygenation". Progress in Oceanography. 145: 1–24. Bibcode:2016PrOce.145....1B. doi:10.1016/j.pocean.2016.04.002.
- ↑ Keeling, Ralph F.; Garcia, Hernan E. (2002). "The change in oceanic O2 inventory associated with recent global warming". Proceedings of the National Academy of Sciences. 99 (12): 7848–7853. Bibcode:2002PNAS...99.7848K. doi:10.1073/pnas.122154899. PMC 122983. PMID 12048249.
- ↑ Pendleton, L.H., Hoegh-Guldberg, O., Langdon, C. and Comte, A. (2016) "Multiple stressors and ecological complexity require a new approach to coral reef research". Frontiers in Marine Science, 3: 36. doi:10.3389/fmars.2016.00036
- ↑ Gruber, Nicolas. "Warming up, turning sour, losing breath: ocean biogeochemistry under global change." Philosophical Transactions of the Royal Society of London A: Mathematical, Physical and Engineering Sciences 369.1943 (2011): 1980–1996.
- ↑ Anthony, et al. (May 2011) "Ocean acidification and warming will lower coral reef resilience." Global Change biology, Volume 17, Issue 5, Pages 1798–1808
- ↑ Goldenberg, Silvan U, et al. (2017) "Boosted food web productivity through ocean acidification collapses under warming." Global Change Biology.
- ↑ Pistevos, Jennifer CA, et al. (2015) "Ocean acidification and global warming impair shark hunting behaviour and growth." Scientific reports 5: 16293.
- 1 2 Österblom, H., Crona, B.I., Folke, C., Nyström, M. and Troell, M. (2017) "Marine ecosystem science on an intertwined planet". Ecosystems, 20(1): 54–61. doi:10.1007/s10021-016-9998-6
- ↑ Robert May forecasts the future New Scientist, 15 November 2006.
- 1 2 Pauly, Daniel (1995) "Anecdotes and the shifting baseline syndrome of fisheries". Trends in ecology & evolution, 10(10): 430.