Mechanotransduction
Mechanotransduction (mechano + transduction) is any of various mechanisms by which cells convert mechanical stimulus into electrochemical activity.[1][2][3][4] This form of sensory transduction is responsible for a number of senses and physiological processes in the body, including proprioception, touch,[5] balance, and hearing.[6][7][8] The basic mechanism of mechanotransduction involves converting mechanical signals into electrical or chemical signals.
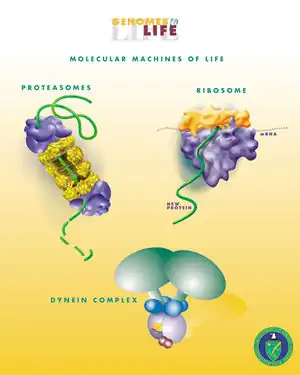
In this process, a mechanically gated ion channel makes it possible for sound, pressure, or movement to cause a change in the excitability of specialized sensory cells and sensory neurons.[9] The stimulation of a mechanoreceptor causes mechanically sensitive ion channels to open and produce a transduction current that changes the membrane potential of the cell.[10] Typically the mechanical stimulus gets filtered in the conveying medium before reaching the site of mechanotransduction.[11] Cellular responses to mechanotransduction are variable and give rise to a variety of changes and sensations. Broader issues involved include molecular biomechanics.
Single-molecule biomechanics studies of proteins and DNA, and mechanochemical coupling in molecular motors have demonstrated the critical importance of molecular mechanics as a new frontier in bioengineering and life sciences. Protein domains, connected by intrinsically disordered flexible linker domains, induce long-range allostery via protein domain dynamics. The resultant dynamic modes cannot be generally predicted from static structures of either the entire protein or individual domains. They can however be inferred by comparing different structures of a protein (as in Database of Molecular Motions). They can also be suggested by sampling in extensive molecular dynamics trajectories[12] and principal component analysis,[13] or they can be directly observed using spectra[14][15] measured by neutron spin echo spectroscopy. Current findings indicate that the mechanotransduction channel in hair cells is a complex biological machine. Mechanotransduction also includes the use of chemical energy to do mechanical work.[16]
Ear
One such mechanism is the opening of ion channels in the hair cells of the cochlea in the inner ear.
Air pressure changes in the ear canal cause the vibrations of the tympanic membrane and middle ear ossicles. At the end of the ossicular chain, movement of the stapes footplate within the oval window of the cochlea, in turn, generates a pressure field within the cochlear fluids, imparting a pressure differential across the basilar membrane. A sinusoidal pressure wave results in localized vibrations of the organ of Corti: near the base for high frequencies, near the apex for low frequencies. The cochlea thus acts as an 'acoustic prism', distributing the energy of each Fourier component (which represent particular frequencies) of a complex sound at different locations along its longitudinal axis. Hair cells in the cochlea are stimulated when the basilar membrane is driven up and down by differences in the fluid pressure between the scala vestibuli and scala tympani. Because this motion is accompanied by a shearing motion between the tectorial membrane and the reticular lamina of the organ of Corti, the hair bundles that link the two are deflected, which initiates mechano-electrical transduction. When the basilar membrane is driven upward, shear between the hair cells and the tectorial membrane deflects hair bundles in the excitatory direction, toward their tall edge. At the midpoint of an oscillation the hair bundles resume their resting position. When the basilar membrane moves downward, the hair bundles are driven in the inhibitory direction.
Basilar Membrane motion causes a shearing motion between the reticular lamina and the tectorial membrane, thereby activating the mechano-sensory apparatus of the hair bundle, which in turn generates a receptor potential in the hair cells.
Thus the sound pressure wave is transduced to an electrical signal which can be processed as sound in higher parts of the auditory system.
Skeletal Muscle
When a deformation is imposed on a muscle, changes in cellular and molecular conformations link the mechanical forces with biochemical signals, and the close integration of mechanical signals with electrical, metabolic, and hormonal signaling may disguise the aspect of the response that is specific to the mechanical forces.[17]
Cartilage
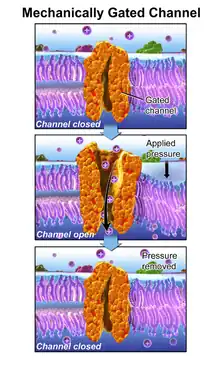
One of the main mechanical functions of articular cartilage is to act as a low-friction, load-bearing surface. Due to its unique location at joint surfaces, articular cartilage experiences a range of static and dynamic forces that include shear, compression and tension. These mechanical loads are absorbed by the cartilage extracellular matrix (ECM), where they are subsequently dissipated and transmitted to chondrocytes (cartilage cells).
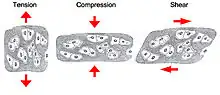
Chondrocytes sense and convert the mechanical signals they receive into biochemical signals, which subsequently direct and mediate both anabolic (matrix building) and catabolic (matrix degrading) processes. These processes include the synthesis of matrix proteins (type II collagen and proteoglycans), proteases, protease inhibitors, transcription factors, cytokines and growth factors.[18][19]
The balance that is struck between anabolic and catabolic processes is strongly influenced by the type of loading that cartilage experiences. High strain rates (such as which occurs during impact loading) cause tissue damage, degradation, decreased matrix production and apoptosis.[20][21] Decreased mechanical loading over long periods, such as during extended bed-rest, causes a loss of matrix production.[22] Static loads have been shown to be detrimental to biosynthesis[23] while oscillatory loads at low frequencies (similar that of a normal walking gait) have been shown to be beneficial in maintaining health and increasing matrix synthesis.[24] Due to the complexity of in-vivo loading conditions and the interplay of other mechanical and biochemical factors, the question of what an optimal loading regimen may be or whether one exists remain unanswered.
Although studies have shown that, like most biological tissues, cartilage is capable of mechanotransduction, the precise mechanisms by which this is done remain unknown. However, there exist a few hypotheses which begin with the identification of mechanoreceptors.
In order for mechanical signals to be sensed, there need to be mechanoreceptors on the surface of chondrocytes. Candidates for chondrocyte mechanoreceptors include stretch-activated ion channels (SAC),[25] the hyaluronan receptor CD44, annexin V (a collagen type II receptor),[26] and integrin receptors (of which there exist several types on chondrocytes).
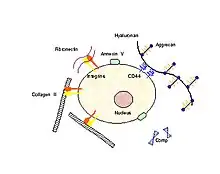
Using the integrin-linked mechanotransduction pathway as an example (being one of the better studied pathways), it has been shown to mediate chondrocyte adhesion to cartilage surfaces,[27] mediate survival signaling[28] and regulate matrix production and degradation.[29]
Integrin receptors have an extracellular domain that binds to the ECM proteins (collagen, fibronectin, laminin, vitronectin and osteopontin), and a cytoplasmic domain that interacts with intracellular signaling molecules. When an integrin receptor binds to its ECM ligand and is activated, additional integrins cluster around the activated site. In addition, kinases (e.g., focal adhesion kinase, FAK) and adapter proteins (e.g., paxillin, Pax, talin, Tal and Shc) are recruited to this cluster, which is called the focal adhesion complex (FAC). The activation of these FAC molecules in turn, triggers downstream events that up-regulate and /or down-regulate intracellular processes such as transcription factor activation and gene regulation resulting in apoptosis or differentiation.
In addition to binding to ECM ligands, integrins are also receptive to autocrine and paracrine signals such as growth factors in the TGF-beta family. Chondrocytes have been shown to secrete TGF-b, and upregulate TGF-b receptors in response to mechanical stimulation; this secretion may be a mechanism for autocrine signal amplification within the tissue.[30]
Integrin signaling is just one example of multiple pathways that are activated when cartilage is loaded. Some intracellular processes that have been observed to occur within these pathways include phosphorylation of ERK1/2, p38 MAPK, and SAPK/ERK kinase-1 (SEK-1) of the JNK pathway[31] as well as changes in cAMP levels, actin re-organization and changes in the expression of genes which regulate cartilage ECM content.[32]
More recent studies have hypothesized that chondrocyte primary cilium act as a mechanoreceptor for the cell, transducing forces from the extracellular matrix into the cell. Each chondrocyte has one cilium and it is hypothesized to transmit mechanical signals by way of bending in response to ECM loading. Integrins have been identified on the upper shaft of the cilium, acting as anchors to the collagen matrix around it.[33] Recent studies published by Wann et al. in FASEB Journal have demonstrated for the first time that primary cilia are required for chondrocyte mechanotransduction. Chondrocytes derived from IFT88 mutant mice did not express primary cilia and did not show the characteristic mechanosensitive up regulation of proteoglycan synthesis seen in wild type cells[34]
It is important to examine the mechanotransduction pathways in chondrocytes since mechanical loading conditions which represent an excessive or injurious response upregulates synthetic activity and increases catabolic signalling cascades involving mediators such as NO and MMPs. In addition, studies by Chowdhury TT and Agarwal S have shown that mechanical loading which represents physiological loading conditions will block the production of catabolic mediators (iNOS, COX-2, NO, PGE2) induced by inflammatory cytokines (IL-1) and restore anabolic activities. Thus an improved understanding of the interplay of biomechanics and cell signalling will help to develop therapeutic methods for blocking catabolic components of the mechanotransduction pathway. A better understanding of the optimal levels of in vivo mechanical forces are therefore necessary for maintaining the health and viability of cartilage, preventative techniques may be devised for the prevention of cartilage degradation and disease.
References
- ↑ Biswas, Abhijit; Manivannan, M.; Srinivasan, Mandyam A. (2015). "Vibrotactile Sensitivity Threshold: Nonlinear Stochastic Mechanotransduction Model of the Pacinian Corpuscle". IEEE Transactions on Haptics. 8 (1): 102–113. doi:10.1109/TOH.2014.2369422. PMID 25398183. S2CID 15326972.
- ↑ Katsumi, A.; Orr, AW; Tzima, E; Schwartz, MA (2003). "Integrins in Mechanotransduction". Journal of Biological Chemistry. 279 (13): 12001–4. doi:10.1074/jbc.R300038200. PMID 14960578.
- ↑ Qin, Y.; Qin, Y; Liu, J; Tanswell, AK; Post, M (1996). "Mechanical Strain Induces pp60src Activation and Translocation to Cytoskeleton in Fetal Rat Lung Cells". Journal of Biological Chemistry. 271 (12): 7066–71. doi:10.1074/jbc.271.12.7066. PMID 8636139.
- ↑ Bidhendi, Amir J; Altartouri, Bara; Gosselin, Frédérick P.; Geitmann, Anja (2019). "Mechanical stress initiates and sustains the morphogenesis of wavy leaf epidermal cells". Cell Reports. 28 (5): 1237–1250. doi:10.1016/j.celrep.2019.07.006. PMID 31365867.
- ↑ Biswas, Abhijit; Manivannan, M.; Srinivasan, Mandyam A. (2014). "Nonlinear two stage mechanotransduction model and neural response of Pacinian Corpuscle". Biomedical Science and Engineering Center Conference (BSEC), 2014 Annual Oak Ridge National Laboratory. USA: IEEE. pp. 1–4. doi:10.1109/BSEC.2014.6867740.
- ↑ Tavernarakis, Nektarios; Driscoll, Monica (1997). "Molecular Modeling of Mechanotransduction in the Nematode Caenorhabditis Elegans". Annual Review of Physiology. 59: 659–89. doi:10.1146/annurev.physiol.59.1.659. PMID 9074782.
- ↑ Howard, J; Roberts, W M; Hudspeth, A J (1988). "Mechanoelectrical Transduction by Hair Cells". Annual Review of Biophysics and Biophysical Chemistry. 17: 99–124. doi:10.1146/annurev.bb.17.060188.000531. PMID 3293600.
- ↑ Hackney, CM; Furness, DN (1995). "Mechanotransduction in vertebrate hair cells: Structure and function of the stereociliary bundle". The American Journal of Physiology. 268 (1 Pt 1): C1–13. doi:10.1152/ajpcell.1995.268.1.C1. PMID 7840137.
- ↑ Gillespie, Peter G.; Walker, Richard G. (2001). "Molecular basis of mechanosensory transduction". Nature. 413 (6852): 194–202. Bibcode:2001Natur.413..194G. doi:10.1038/35093011. PMID 11557988. S2CID 4388399.
- ↑ Grigg, P (1986). "Biophysical studies of mechanoreceptors". Journal of Applied Physiology. 60 (4): 1107–15. doi:10.1152/jappl.1986.60.4.1107. PMID 2422151.
- ↑ Biswas, Abhijit; Manivannan, M.; Srinivasan, Mandyam A. (2015). "Multiscale Layered Biomechanical Model of the Pacinian Corpuscle". IEEE Transactions on Haptics. 8 (1): 31–42. doi:10.1109/TOH.2014.2369416. PMID 25398182. S2CID 24658742.
- ↑ Potestio R, Pontiggia F, Micheletti C (Jun 2009). "Coarse-grained description of protein internal dynamics: an optimal strategy for decomposing proteins in rigid subunits". Biophysical Journal. 96 (12): 4993–5002. Bibcode:2009BpJ....96.4993P. doi:10.1016/j.bpj.2009.03.051. PMC 2712024. PMID 19527659.
- ↑ Baron R, Vellore NA (Jul 2012). "LSD1/CoREST is an allosteric nanoscale clamp regulated by H3-histone-tail molecular recognition". Proceedings of the National Academy of Sciences of the United States of America. 109 (31): 12509–14. Bibcode:2012PNAS..10912509B. doi:10.1073/pnas.1207892109. PMC 3411975. PMID 22802671.
- ↑ Farago B, Li J, Cornilescu G, Callaway DJ, Bu Z (Nov 2010). "Activation of nanoscale allosteric protein domain motion revealed by neutron spin echo spectroscopy". Biophysical Journal. 99 (10): 3473–3482. Bibcode:2010BpJ....99.3473F. doi:10.1016/j.bpj.2010.09.058. PMC 2980739. PMID 21081097.
- ↑ Bu Z, Biehl R, Monkenbusch M, Richter D, Callaway DJ (Dec 2005). "Coupled protein domain motion in Taq polymerase revealed by neutron spin-echo spectroscopy". Proceedings of the National Academy of Sciences of the United States of America. 102 (49): 17646–17651. Bibcode:2005PNAS..10217646B. doi:10.1073/pnas.0503388102. PMC 1345721. PMID 16306270.
- ↑ Nakano, Tadashi; Eckford, Andrew W.; Haraguchi, Tokuko (12 September 2013). Molecular Communication. Cambridge University Press. ISBN 978-1-107-02308-6.
- ↑ Burkholder, TJ (2007). "Mechanotransduction in skeletal muscle". Frontiers in Bioscience. 12: 174–91. doi:10.2741/2057. PMC 2043154. PMID 17127292.
- ↑ Fitzgerald, J. B.; Jin, M; Dean, D; Wood, DJ; Zheng, MH; Grodzinsky, AJ (2004). "Mechanical Compression of Cartilage Explants Induces Multiple Time-dependent Gene Expression Patterns and Involves Intracellular Calcium and Cyclic AMP". Journal of Biological Chemistry. 279 (19): 19502–11. doi:10.1074/jbc.M400437200. PMID 14960571.
- ↑ Fitzgerald, J. B.; Jin, M; Grodzinsky, AJ (2006). "Shear and Compression Differentially Regulate Clusters of Functionally Related Temporal Transcription Patterns in Cartilage Tissue". Journal of Biological Chemistry. 281 (34): 24095–103. doi:10.1074/jbc.M510858200. PMID 16782710.
- ↑ Kurz, Bodo; Jin, Moonsoo; Patwari, Parth; Cheng, Debbie M.; Lark, Michael W.; Grodzinsky, Alan J. (2001). "Biosynthetic response and mechanical properties of articular cartilage after injurious compression". Journal of Orthopaedic Research. 19 (6): 1140–6. doi:10.1016/S0736-0266(01)00033-X. PMID 11781016.
- ↑ Loening, A; James, IE; Levenston, ME; Badger, AM; Frank, EH; Kurz, B; Nuttall, ME; Hung, HH; Blake, SM (2000). "Injurious Mechanical Compression of Bovine Articular Cartilage Induces Chondrocyte Apoptosis". Archives of Biochemistry and Biophysics. 381 (2): 205–12. doi:10.1006/abbi.2000.1988. PMID 11032407. S2CID 21964244.
- ↑ Behrens, Fred; Kraft, Ellen L.; Oegema, Theodore R. (1989). "Biochemical changes in articular cartilage after joint immobilization by casting or external fixation". Journal of Orthopaedic Research. 7 (3): 335–43. doi:10.1002/jor.1100070305. PMID 2703926. S2CID 34651862.
- ↑ Torzilli, P. A.; Deng, X-H.; Ramcharan, M. (2006). "Effect of Compressive Strain on Cell Viability in Statically Loaded Articular Cartilage". Biomechanics and Modeling in Mechanobiology. 5 (2–3): 123–32. doi:10.1007/s10237-006-0030-5. PMID 16506016. S2CID 39216430.
- ↑ Sah, Robert L.-Y.; Kim, Young-Jo; Doong, Joe-Yuan H.; Grodzinsky, Alan J.; Plass, Anna H. K.; Sandy, John D. (1989). "Biosynthetic response of cartilage explants to dynamic compression". Journal of Orthopaedic Research. 7 (5): 619–36. doi:10.1002/jor.1100070502. PMID 2760736. S2CID 1933220.
- ↑ Mouw, J. K.; Imler, S. M.; Levenston, M. E. (2006). "Ion-channel Regulation of Chondrocyte Matrix Synthesis in 3D Culture Under Static and Dynamic Compression". Biomechanics and Modeling in Mechanobiology. 6 (1–2): 33–41. doi:10.1007/s10237-006-0034-1. PMID 16767453. S2CID 7270995.
- ↑ Von Der Mark, K.; Mollenhauer, J. (1997). "Annexin V interactions with collagen". Cellular and Molecular Life Sciences. 53 (6): 539–45. doi:10.1007/s000180050069. PMID 9230933. S2CID 21313045.
- ↑ Kurtis, Melissa S.; Tu, Buu P.; Gaya, Omar A.; Mollenhauer, Jürgen; Knudson, Warren; Loeser, Richard F.; Knudson, Cheryl B.; Sah, Robert L. (2001). "Mechanisms of chondrocyte adhesion to cartilage: Role of β1-integrins, CD44, and annexin V". Journal of Orthopaedic Research. 19 (6): 1122–30. doi:10.1016/S0736-0266(01)00051-1. PMID 11781014.
- ↑ Pulai, Judit I.; Del Carlo, Marcello; Loeser, Richard F. (2002). "The ?5?1 integrin provides matrix survival signals for normal and osteoarthritic human articular chondrocytes in vitro". Arthritis & Rheumatism. 46 (6): 1528–35. doi:10.1002/art.10334. PMID 12115183.
- ↑ Millward–Sadler, S. J.; Wright, M. O.; Davies, L. W.; Nuki, G.; Salter, D. M. (2000). "Mechanotransduction via integrins and interleukin–4 results in altered aggrecan and matrix metalloproteinase 3 gene expression in normal, but not osteoarthritic, human articular chondrocytes". Arthritis & Rheumatism. 43 (9): 2091–2099. doi:10.1002/1529-0131(200009)43:9<2091::AID-ANR21>3.0.CO;2-C. PMID 11014361.
- ↑ Millward-Sadler, S. J.; Salter, D. M. (2004). "Integrin-Dependent Signal Cascades in Chondrocyte Mechanotransduction". Annals of Biomedical Engineering. 32 (3): 435–46. doi:10.1023/B:ABME.0000017538.72511.48. PMID 15095818. S2CID 1717838.
- ↑ Fanning, P. J.; Emkey, G; Smith, RJ; Grodzinsky, AJ; Szasz, N; Trippel, SB (2003). "Mechanical Regulation of Mitogen-activated Protein Kinase Signaling in Articular Cartilage". Journal of Biological Chemistry. 278 (51): 50940–8. doi:10.1074/jbc.M305107200. PMID 12952976.
- ↑ Urban, J. P. G. (1994). "The Chondrocyte: A Cell Under Pressure". Rheumatology. 33 (10): 901–908. doi:10.1093/rheumatology/33.10.901. PMID 7921748.
- ↑ McGlashan, S. R.; Jensen, CG; Poole, CA (2006). "Localization of Extracellular Matrix Receptors on the Chondrocyte Primary Cilium". Journal of Histochemistry and Cytochemistry. 54 (9): 1005–14. doi:10.1369/jhc.5A6866.2006. PMID 16651393.
- ↑ Wann, AK; Zuo, N; Haycraft, CJ; et al. (April 2012). "Primary cilia mediate mechanotransduction through control of ATP-induced Ca2+ signaling in compressed chondrocytes". FASEB J. 26 (4): 1663–71. doi:10.1096/fj.11-193649. PMC 3316893. PMID 22223751.
Further reading
- Khan, K M; Scott, A (2009). "Mechanotherapy: How physical therapists' prescription of exercise promotes tissue repair". British Journal of Sports Medicine. 43 (4): 247–52. doi:10.1136/bjsm.2008.054239. PMC 2662433. PMID 19244270.
- Mammano, F.; Nobili, R (1993). "Biophysics of the cochlea: Linear approximation". The Journal of the Acoustical Society of America. 93 (6): 3320–32. Bibcode:1993ASAJ...93.3320M. doi:10.1121/1.405716. PMID 8326060.
- v. Békésy, Georg (1952). "DC Resting Potentials Inside the Cochlear Partition". The Journal of the Acoustical Society of America. 24 (1): 72–76. Bibcode:1952ASAJ...24...72V. doi:10.1121/1.1906851.
- 1. Kandel, E.R., Schwartz, J.H., Jessell, T.M., Principles of Neural Science. New York: McGraw-Hill ed, ed. 4th. 2000.
- Hudspeth, A. J.; Choe, Y.; Mehta, A. D.; Martin, P. (2000). "Putting ion channels to work: Mechanoelectrical transduction, adaptation, and amplification by hair cells". Proceedings of the National Academy of Sciences. 97 (22): 11765–72. Bibcode:2000PNAS...9711765H. doi:10.1073/pnas.97.22.11765. PMC 34347. PMID 11050207.
- Hudspeth, A. J. (1989). "How the ear's works work". Nature. 341 (6241): 397–404. Bibcode:1989Natur.341..397H. doi:10.1038/341397a0. PMID 2677742. S2CID 33117543.
External links
- www.du.edu/~kinnamon/3640/hearing/hearing.html
- Cellular+Mechanotransduction at the US National Library of Medicine Medical Subject Headings (MeSH)