Biodegradation
Biodegradation is the breakdown of organic matter by microorganisms, such as bacteria and fungi.[lower-alpha 1][2] It is generally assumed to be a natural process, which differentiates it from composting. Composting is a human-driven process in which biodegradation occurs under a specific set of circumstances.

The process of biodegradation is threefold: first an object undergoes biodeterioration, which is the mechanical weakening of its structure; then follows biofragmentation, which is the breakdown of materials by microorganisms; and finally assimilation, which is the incorporation of the old material into new cells.
In practice, almost all chemical compounds and materials are subject to biodegradation, the key element being time. Things like vegetables may degrade within days, while glass and some plastics take many millennia to decompose. A standard for biodegradability used by the European Union is that greater than 90% of the original material must be converted into CO2, water and minerals by biological processes within 6 months.
Mechanisms
The process of biodegradation can be divided into three stages: biodeterioration, biofragmentation, and assimilation.[3] Biodeterioration is sometimes described as a surface-level degradation that modifies the mechanical, physical and chemical properties of the material. This stage occurs when the material is exposed to abiotic factors in the outdoor environment and allows for further degradation by weakening the material's structure. Some abiotic factors that influence these initial changes are compression (mechanical), light, temperature and chemicals in the environment.[3] While biodeterioration typically occurs as the first stage of biodegradation, it can in some cases be parallel to biofragmentation.[4] Hueck,[5] however, defined Biodeterioration as the undesirable action of living organisms on Man's materials, involving such things as breakdown of stone facades of buildings,[6] corrosion of metals by microorganisms or merely the esthetic changes induced on man-made structures by the growth of living organisms.[6]
Biofragmentation of a polymer is the lytic process in which bonds within a polymer are cleaved, generating oligomers and monomers in its place.[3] The steps taken to fragment these materials also differ based on the presence of oxygen in the system. The breakdown of materials by microorganisms when oxygen is present is aerobic digestion, and the breakdown of materials when oxygen is not present is anaerobic digestion.[7] The main difference between these processes is that anaerobic reactions produce methane, while aerobic reactions do not (however, both reactions produce carbon dioxide, water, some type of residue, and a new biomass).[8] In addition, aerobic digestion typically occurs more rapidly than anaerobic digestion, while anaerobic digestion does a better job reducing the volume and mass of the material.[7] Due to anaerobic digestion's ability to reduce the volume and mass of waste materials and produce a natural gas, anaerobic digestion technology is widely used for waste management systems and as a source of local, renewable energy.[9]
In the assimilation stage, the resulting products from biofragmentation are then integrated into microbial cells.[3] Some of the products from fragmentation are easily transported within the cell by membrane carriers. However, others still have to undergo biotransformation reactions to yield products that can then be transported inside the cell. Once inside the cell, the products enter catabolic pathways that either lead to the production of adenosine triphosphate (ATP) or elements of the cells structure.[3]
- Aerobic biodegradation equation
- Cpolymer + O2 → Cresidue + Cbiomass + CO2 + H2O
- Anaerobic biodegradation equation
- Cpolymer → Cresidue + Cbiomass + CO2 + CH4 + H2O
Factors affecting biodegradation rate

In practice, almost all chemical compounds and materials are subject to biodegradation processes. The significance, however, is in the relative rates of such processes, such as days, weeks, years or centuries. A number of factors determine the rate at which this degradation of organic compounds occurs. Factors include light, water, oxygen and temperature.[10] The degradation rate of many organic compounds is limited by their bioavailability, which is the rate at which a substance is absorbed into a system or made available at the site of physiological activity,[11] as compounds must be released into solution before organisms can degrade them. The rate of biodegradation can be measured in a number of ways. Respirometry tests can be used for aerobic microbes. First one places a solid waste sample in a container with microorganisms and soil, and then aerates the mixture. Over the course of several days, microorganisms digest the sample bit by bit and produce carbon dioxide – the resulting amount of CO2 serves as an indicator of degradation. Biodegradability can also be measured by anaerobic microbes and the amount of methane or alloy that they are able to produce.[12]
It's important to note factors that affect biodegradation rates during product testing to ensure that the results produced are accurate and reliable. Several materials will test as being biodegradable under optimal conditions in a lab for approval but these results may not reflect real world outcomes where factors are more variable.[13] For example, a material may have tested as biodegrading at a high rate in the lab may not degrade at a high rate in a landfill because landfills often lack light, water, and microbial activity that are necessary for degradation to occur.[14] Thus, it is very important that there are standards for plastic biodegradable products, which have a large impact on the environment. The development and use of accurate standard test methods can help ensure that all plastics that are being produced and commercialized will actually biodegrade in natural environments.[15] One test that has been developed for this purpose is DINV 54900.[16]
Product | Time to Biodegrade |
---|---|
Paper towel | 2–4 weeks |
Newspaper | 6 weeks |
Apple core | 2 months |
Cardboard box | 2 months |
Wax coated milk carton | 3 months |
Cotton gloves | 1–5 months |
Wool gloves | 1 year |
Plywood | 1–3 years |
Painted wooden sticks | 13 years |
Plastic bags | 10–20 years |
Tin cans | 50 years |
Disposable diapers | 50–100 years |
Plastic bottle | 100 years |
Aluminium cans | 200 years |
Glass bottles | Undetermined |
Vegetables | 5 days – 1 month |
Paper | 2–5 months |
Cotton T-shirt | 6 months |
Orange peels | 6 months |
Tree leaves | 1 year |
Wool socks | 1–5 years |
Plastic-coated paper milk cartons | 5 years |
Leather shoes | 25–40 years |
Nylon fabric | 30–40 years |
Tin cans | 50–100 years |
Aluminium cans | 80–100 years |
Glass bottles | 1 million years |
Styrofoam cup | 500 years to forever |
Plastic bags | 500 years to forever |
Plastics
The term Biodegradable Plastics refers to materials that maintain their mechanical strength during practical use but break down into low-weight compounds and non-toxic byproducts after their use.[18] This breakdown is made possible through an attack of microorganisms on the material, which is typically a non-water-soluble polymer.[4] Such materials can be obtained through chemical synthesis, fermentation by microorganisms, and from chemically modified natural products.[19]
Plastics biodegrade at highly variable rates. PVC-based plumbing is selected for handling sewage because PVC resists biodegradation. Some packaging materials on the other hand are being developed that would degrade readily upon exposure to the environment.[20] Examples of synthetic polymers that biodegrade quickly include polycaprolactone, other polyesters and aromatic-aliphatic esters, due to their ester bonds being susceptible to attack by water. A prominent example is poly-3-hydroxybutyrate, the renewably derived polylactic acid. Others are the cellulose-based cellulose acetate and celluloid (cellulose nitrate).
- Polylactic acid is an example of a plastic that biodegrades quickly.
Under low oxygen conditions plastics break down more slowly. The breakdown process can be accelerated in specially designed compost heap. Starch-based plastics will degrade within two to four months in a home compost bin, while polylactic acid is largely undecomposed, requiring higher temperatures.[21] Polycaprolactone and polycaprolactone-starch composites decompose slower, but the starch content accelerates decomposition by leaving behind a porous, high surface area polycaprolactone. Nevertheless, it takes many months.[22]
In 2016, a bacterium named Ideonella sakaiensis was found to biodegrade PET. In 2020, the PET degrading enzyme of the bacterium, PETase, has been genetically modified and combined with MHETase to break down PET faster, and also degrade PEF.[23][24][25] In 2021, researchers reported that a mix of microorganisms from cow stomachs could break down three types of plastics.[26][27]
Many plastic producers have gone so far even to say that their plastics are compostable, typically listing corn starch as an ingredient. However, these claims are questionable because the plastics industry operates under its own definition of compostable:
- "that which is capable of undergoing biological decomposition in a compost site such that the material is not visually distinguishable and breaks down into carbon dioxide, water, inorganic compounds and biomass at a rate consistent with known compostable materials." (Ref: ASTM D 6002)[28]
The term "composting" is often used informally to describe the biodegradation of packaging materials. Legal definitions exist for compostability, the process that leads to compost. Four criteria are offered by the European Union:[29][30]
- Chemical composition: volatile matter and heavy metals as well as fluorine should be limited.
- Biodegradability: the conversion of >90% of the original material into CO2, water and minerals by biological processes within 6 months.
- Disintegrability: at least 90% of the original mass should be decomposed into particles that are able to pass through a 2x2 mm sieve.
- Quality: absence of toxic substances and other substances that impede composting.
Biodegradable technology
Biodegradable technology is established technology with some applications in product packaging, production, and medicine.[31] The chief barrier to widespread implementation is the trade-off between biodegradability and performance. For example, lactide-based plastics are inferior packaging properties in comparison to traditional materials.
Oxo-biodegradation is defined by CEN (the European Standards Organisation) as "degradation resulting from oxidative and cell-mediated phenomena, either simultaneously or successively." While sometimes described as "oxo-fragmentable," and "oxo-degradable" these terms describe only the first or oxidative phase and should not be used for material which degrades by the process of oxo-biodegradation defined by CEN: the correct description is "oxo-biodegradable." Oxo-biodegradable formulations accelerate the biodegradation process but it takes considerable skill and experience to balance the ingredients within the formulations so as to provide the product with a useful life for a set period, followed by degradation and biodegradation.[32]
Biodegradable technology is especially utilized by the bio-medical community. Biodegradable polymers are classified into three groups: medical, ecological, and dual application, while in terms of origin they are divided into two groups: natural and synthetic.[18] The Clean Technology Group is exploiting the use of supercritical carbon dioxide, which under high pressure at room temperature is a solvent that can use biodegradable plastics to make polymer drug coatings. The polymer (meaning a material composed of molecules with repeating structural units that form a long chain) is used to encapsulate a drug prior to injection in the body and is based on lactic acid, a compound normally produced in the body, and is thus able to be excreted naturally. The coating is designed for controlled release over a period of time, reducing the number of injections required and maximizing the therapeutic benefit. Professor Steve Howdle states that biodegradable polymers are particularly attractive for use in drug delivery, as once introduced into the body they require no retrieval or further manipulation and are degraded into soluble, non-toxic by-products. Different polymers degrade at different rates within the body and therefore polymer selection can be tailored to achieve desired release rates.[33]
Other biomedical applications include the use of biodegradable, elastic shape-memory polymers. Biodegradable implant materials can now be used for minimally invasive surgical procedures through degradable thermoplastic polymers. These polymers are now able to change their shape with increase of temperature, causing shape memory capabilities as well as easily degradable sutures. As a result, implants can now fit through small incisions, doctors can easily perform complex deformations, and sutures and other material aides can naturally biodegrade after a completed surgery.[34]
Biodegradation vs. composting
There is no universal definition for biodegradation and there are various definitions of composting, which has led to much confusion between the terms. They are often lumped together; however, they do not have the same meaning. Biodegradation is the naturally-occurring breakdown of materials by microorganisms such as bacteria and fungi or other biological activity.[35] Composting is a human-driven process in which biodegradation occurs under a specific set of circumstances.[36] The predominant difference between the two is that one process is naturally-occurring and one is human-driven.
Biodegradable material is capable of decomposing without an oxygen source (anaerobically) into carbon dioxide, water, and biomass, but the timeline is not very specifically defined. Similarly, compostable material breaks down into carbon dioxide, water, and biomass; however, compostable material also breaks down into inorganic compounds. The process for composting is more specifically defined, as it is controlled by humans. Essentially, composting is an accelerated biodegradation process due to optimized circumstances.[37] Additionally, the end product of composting not only returns to its previous state, but also generates and adds beneficial microorganisms to the soil called humus. This organic matter can be used in gardens and on farms to help grow healthier plants in the future.[38] Composting more consistently occurs within a shorter time frame since it is a more defined process and is expedited by human intervention. Biodegradation can occur in different time frames under different circumstances, but is meant to occur naturally without human intervention.
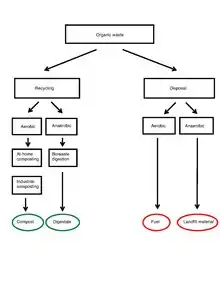
Even within composting, there are different circumstances under which this can occur. The two main types of composting are at-home versus commercial. Both produce healthy soil to be reused - the main difference lies in what materials are able to go into the process.[37] At-home composting is mostly used for food scraps and excess garden materials, such as weeds. Commercial composting is capable of breaking down more complex plant-based products, such as corn-based plastics and larger pieces of material, like tree branches. Commercial composting begins with a manual breakdown of the materials using a grinder or other machine to initiate the process. Because at-home composting usually occurs on a smaller scale and does not involve large machinery, these materials would not fully decompose in at-home composting. Furthermore, one study has compared and contrasted home and industrial composting, concluding that there are advantages and disadvantages to both.[40]
The following studies provide examples in which composting has been defined as a subset of biodegradation in a scientific context. The first study, "Assessment of Biodegradability of Plastics Under Simulated Composting Conditions in a Laboratory Test Setting," clearly examines composting as a set of circumstances that falls under the category of degradation.[41] Additionally, this next study looked at the biodegradation and composting effects of chemically and physically crosslinked polylactic acid.[42] Notably discussing composting and biodegrading as two distinct terms. The third and final study reviews European standardization of biodegradable and compostable material in the packaging industry, again using the terms separately.[43]
The distinction between these terms is crucial because waste management confusion leads to improper disposal of materials by people on a daily basis. Biodegradation technology has led to massive improvements in how we dispose of waste; there now exist trash, recycling, and compost bins in order to optimize the disposal process. However, if these waste streams are commonly and frequently confused, then the disposal process is not at all optimized.[44] Biodegradable and compostable materials have been developed to ensure more of human waste is able to breakdown and return to its previous state, or in the case of composting even add nutrients to the ground.[45] When a compostable product is thrown out as opposed to composted and sent to a landfill, these inventions and efforts are wasted. Therefore, it is important for citizens to understand the difference between these terms so that materials can be disposed of properly and efficiently.
Environmental and social effects
Plastic pollution from illegal dumping poses health risks to wildlife. Animals often mistake plastics for food, resulting in intestinal entanglement. Slow-degrading chemicals, like polychlorinated biphenyls (PCBs), nonylphenol (NP), and pesticides also found in plastics, can release into environments and subsequently also be ingested by wildlife.[46]
These chemicals also play a role in human health, as consumption of tainted food (in processes called biomagnification and bioaccumulation) has been linked to issues such as cancers,[47] neurological dysfunction,[48] and hormonal changes. A well-known example of biomagnification impacting health in recent times is the increased exposure to dangerously high levels of mercury in fish, which can affect sex hormones in humans.[49]
In efforts to remediate the damages done by slow-degrading plastics, detergents, metals, and other pollutants created by humans, economic costs have become a concern. Marine litter in particular is notably difficult to quantify and review.[50] Researchers at the World Trade Institute estimate that cleanup initiatives' cost (specifically in ocean ecosystems) has hit close to thirteen billion dollars a year.[51] The main concern stems from marine environments, with the biggest cleanup efforts centering around garbage patches in the ocean. In 2017, a garbage patch the size of Mexico was found in the Pacific Ocean. It is estimated to be upwards of a million square miles in size. While the patch contains more obvious examples of litter (plastic bottles, cans, and bags), tiny microplastics are nearly impossible to clean up.[52] National Geographic reports that even more non-biodegradable materials are finding their way into vulnerable environments - nearly thirty-eight million pieces a year.[53]
Materials that have not degraded can also serve as shelter for invasive species, such as tube worms and barnacles. When the ecosystem changes in response to the invasive species, resident species and the natural balance of resources, genetic diversity, and species richness is altered.[54] These factors may support local economies in way of hunting and aquaculture, which suffer in response to the change.[55] Similarly, coastal communities which rely heavily on ecotourism lose revenue thanks to a buildup of pollution, as their beaches or shores are no longer desirable to travelers. The World Trade Institute also notes that the communities who often feel most of the effects of poor biodegradation are poorer countries without the means to pay for their cleanup.[51] In a positive feedback loop effect, they in turn have trouble controlling their own pollution sources.[56]
Etymology of "biodegradable"
The first known use of biodegradable in a biological context was in 1959 when it was employed to describe the breakdown of material into innocuous components by microorganisms.[57] Now biodegradable is commonly associated with environmentally friendly products that are part of the earth's innate cycles like the carbon cycle and capable of decomposing back into natural elements.
See also
- Anaerobic digestion
- Assimilation (biology)
- Bioaccumulation
- Biodegradability prediction
- Biodegradable electronics
- Biodegradable polythene film
- Biodegradation (journal)
- Biomagnification
- Bioplastic – biodegradable, bio-based plastics
- Bioremediation
- Decomposition – reduction of the body of a formerly living organism into simpler forms of matter
- Landfill gas monitoring
- List of environment topics
- Microbial biodegradation
- Photodegradation
Notes
References
- Vert M, Doi Y, Hellwich KH, Hess M, Hodge P, Kubisa P, Rinaudo M, Schué F (2012). "Terminology for biorelated polymers and applications (IUPAC Recommendations 2012)". Pure and Applied Chemistry. 84 (2): 377–410. doi:10.1351/PAC-REC-10-12-04. S2CID 98107080.
- Focht DD. "Biodegradation". AccessScience. doi:10.1036/1097-8542.422025.
- Lucas N, Bienaime C, Belloy C, Queneudec M, Silvestre F, Nava-Saucedo JE (September 2008). "Polymer biodegradation: mechanisms and estimation techniques". Chemosphere. 73 (4): 429–42. Bibcode:2008Chmsp..73..429L. doi:10.1016/j.chemosphere.2008.06.064. PMID 18723204.
- Muller R (2005). "Biodegradability of Polymers: Regulations and Methods for Testing" (PDF). In Steinbüchel A (ed.). Biopolymers. Wiley-VCH. doi:10.1002/3527600035.bpola012. ISBN 978-3-527-30290-1. Archived from the original (PDF) on 2018-09-19. Retrieved 2018-09-19.
- Hueck, Hans (January 1966). "The biodeterioration of materials as part of hylobiology". Material und Organismen. 1: 5–34 – via ISSN 00255270.
- Allsopp, Dennis (2004). Introduction to Biodeterioration. Cambridge: Cambridge University Press. ISBN 9780511617065.
- "Aerobic and Anaerobic Biodegradation" (PDF). Fundamentals of Aerobic & Anaerobic Biodegradation Process. Polimernet Plastik San. Tic. Ltd. Şti. Archived (PDF) from the original on 2011-04-19.
- Van der Zee M (2011). "Analytical Methods for Monitoring Biodegradation Processes of Environmentally Degradable Polymers". Archived from the original on 2019-02-18. Retrieved 2019-01-21.
- Klinkner BA (2014). "Anaerobic Digestion as a Renewable Energy Source and Waste Management Technology: What Must be Done for this Technology to Realize Success in the United States?". University of Massachusetts Law Review. 9: 68–96. Archived from the original on 2020-06-29. Retrieved 2018-09-23.
- Haider T, Völker C, Kramm J, Landfester K, Wurm FR (July 2018). "Plastics of the future? The impact of biodegradable polymers on the environment and on society". Angewandte Chemie International Edition in English. 58 (1): 50–62. doi:10.1002/anie.201805766. PMID 29972726.
- "Definition of BIOAVAILABILITY". www.merriam-webster.com. Archived from the original on 2018-09-19. Retrieved 2018-09-19.
- Jessop A (2015-09-16). "How is biodegradability measured?". Commercial Waste. Archived from the original on 2018-09-19. Retrieved 2018-09-19.
- Adamcova D, Radziemska M, Fronczyk J, Zloch J, Vaverkova MD (2017). "Research of the biodegradability of degradable/biodegradable plastic material in various types of environments". Przegląd Naukowy. Inżynieria i Kształtowanie Środowiska. 26: 3–14. doi:10.22630/PNIKS.2017.26.1.01.
- "Measuring biodegradability". Science Learning Hub. Archived from the original on 2018-09-19. Retrieved 2018-09-19.
- Scott G, Gilead D, eds. (1995). Degradable Polymers. Netherlands: Dordrecht Springer. doi:10.1007/978-94-011-0571-2. ISBN 978-94-010-4253-6.
- Witt U, Yamamoto M, Seeliger U, Müller RJ, Warzelhan V (May 1999). "Biodegradable Polymeric Materials-Not the Origin but the Chemical Structure Determines Biodegradability". Angewandte Chemie. 38 (10): 1438–1442. doi:10.1002/(sici)1521-3773(19990517)38:10<1438::aid-anie1438>3.0.co;2-u. PMID 29711570.
- "Marine Debris Biodegradation Time Line" Archived 2011-11-05 at the Wayback Machine. C-MORE, citing Mote Marine Laboratory, 1993.
- Ikada Y, Tsuji H (February 2000). "Biodegradable polyesters for medical and ecological applications" (PDF). Macromolecular Rapid Communications. 21 (3): 117–132. doi:10.1002/(sici)1521-3927(20000201)21:3<117::aid-marc117>3.0.co;2-x. Archived (PDF) from the original on 2016-03-05. Retrieved 2011-03-08.
- Flieger M, Kantorová M, Prell A, Rezanka T, Votruba J (January 2003). "Biodegradable plastics from renewable sources". Folia Microbiologica. 48 (1): 27–44. doi:10.1007/bf02931273. PMID 12744074. S2CID 32800851.
- Kyrikou I, Briassoulis D (12 Apr 2007). "Biodegradation of Agricultural Plastic Films: A Critical Review". Journal of Polymers and the Environment. 15 (2): 125–150. doi:10.1007/s10924-007-0053-8. S2CID 195331133.
- "Section 6: Biodegradability of Packaging Waste" (PDF). Www3.imperial.ac.uk. Archived (PDF) from the original on 2013-06-02. Retrieved 2014-03-02.
- Wu C (January 2003). "Physical properties and biodegradability of maleated-polycaprolactone/starch composite" (PDF). Polymer Degradation and Stability. 80 (1): 127–134. CiteSeerX 10.1.1.453.4220. doi:10.1016/S0141-3910(02)00393-2. Archived from the original (PDF) on 2016-03-04. Retrieved 2012-06-23.
- Carrington, Damian (28 September 2020). "New super-enzyme eats plastic bottles six times faster". The Guardian. Archived from the original on 12 October 2020. Retrieved 12 October 2020.
- "Plastic-eating enzyme 'cocktail' heralds new hope for plastic waste". phys.org. Archived from the original on 11 October 2020. Retrieved 12 October 2020.
- Knott, Brandon C.; Erickson, Erika; Allen, Mark D.; Gado, Japheth E.; Graham, Rosie; Kearns, Fiona L.; Pardo, Isabel; Topuzlu, Ece; Anderson, Jared J.; Austin, Harry P.; Dominick, Graham; Johnson, Christopher W.; Rorrer, Nicholas A.; Szostkiewicz, Caralyn J.; Copié, Valérie; Payne, Christina M.; Woodcock, H. Lee; Donohoe, Bryon S.; Beckham, Gregg T.; McGeehan, John E. (24 September 2020). "Characterization and engineering of a two-enzyme system for plastics depolymerization". Proceedings of the National Academy of Sciences. 117 (41): 25476–25485. Bibcode:2020PNAS..11725476K. doi:10.1073/pnas.2006753117. ISSN 0027-8424. PMC 7568301. PMID 32989159.
- Spary, Sara. "Cows' stomachs can break down plastic, study finds". CNN. Archived from the original on 14 August 2021. Retrieved 14 August 2021.
- Quartinello, Felice; Kremser, Klemens; Schoen, Herta; Tesei, Donatella; Ploszczanski, Leon; Nagler, Magdalena; Podmirseg, Sabine M.; Insam, Heribert; Piñar, Guadalupe; Sterflingler, Katja; Ribitsch, Doris; Guebitz, Georg M. (2021). "Together Is Better: The Rumen Microbial Community as Biological Toolbox for Degradation of Synthetic Polyesters". Frontiers in Bioengineering and Biotechnology. 9. doi:10.3389/fbioe.2021.684459. ISSN 2296-4185.
- "Compostable". Compostable.info. Archived from the original on 2020-11-12. Retrieved 2014-03-02.
- "Requirements of the EN 13432 standard" (PDF). European Bioplastics. Brussels, Belgium. April 2015. Archived (PDF) from the original on 2018-09-24. Retrieved July 22, 2017.
- Breulmann M, Künkel A, Philipp S, Reimer V, Siegenthaler KO, Skupin G, Yamamoto M (2012). "Polymers, Biodegradable". Ullmann's Encyclopedia of Industrial Chemistry. Weinheim: Wiley-VCH. doi:10.1002/14356007.n21_n01. ISBN 978-3527306732.
- Gross RA, Kalra B (August 2002). "Biodegradable polymers for the environment". Science. 297 (5582): 803–7. Bibcode:2002Sci...297..803G. doi:10.1126/science.297.5582.803. PMID 12161646. Archived from the original on 2020-07-25. Retrieved 2019-06-27.
- Agamuthu P, Faizura PN (April 2005). "Biodegradability of degradable plastic waste". Waste Management & Research. 23 (2): 95–100. doi:10.1177/0734242X05051045. PMID 15864950. S2CID 2552973.
- The University of Nottingham (September 13, 2007). "Using Green Chemistry to Deliver Cutting Edge Drugs". Science Daily. Archived from the original on September 24, 2018. Retrieved September 24, 2018.
- Lendlein A, Langer R (May 2002). "Biodegradable, elastic shape-memory polymers for potential biomedical applications". Science. 296 (5573): 1673–6. Bibcode:2002Sci...296.1673L. doi:10.1126/science.1066102. PMID 11976407. S2CID 21801034.
- Gómez EF, Michel FC (December 2013). "Biodegradability of conventional and bio-based plastics and natural fiber composites during composting, anaerobic digestion and long-term soil incubation". Polymer Degradation and Stability. 98 (12): 2583–2591. doi:10.1016/j.polymdegradstab.2013.09.018.
- "Biodegradable Products Institute - Composting". bpiworld.org. Archived from the original on 2018-09-24. Retrieved 2018-09-24.
- Magdoff F (November 1993). "Building Soils for Better Crops". Soil Science. 156 (5): 371. Bibcode:1993SoilS.156..371M. doi:10.1097/00010694-199311000-00014.
- Morris S, Martin JP. "Humus". AccessScience. doi:10.1036/1097-8542.325510. S2CID 242577363. Archived from the original on 2018-09-24. Retrieved 2018-09-24.
- Kranert M, Behnsen A, Schultheis A, Steinbach D (2002). "Composting in the Framework of the EU Landfill Directive". Microbiology of Composting. Springer Berlin Heidelberg. pp. 473–486. doi:10.1007/978-3-662-08724-4_39. ISBN 9783642087059.
- Martínez-Blanco J, Colón J, Gabarrell X, Font X, Sánchez A, Artola A, Rieradevall J (June 2010). "The use of life cycle assessment for the comparison of biowaste composting at home and full scale". Waste Management (Submitted manuscript). 30 (6): 983–94. Bibcode:2010WaMan..30..983M. doi:10.1016/j.wasman.2010.02.023. PMID 20211555. Archived from the original on 2019-04-01. Retrieved 2018-09-27.
- Starnecker A, Menner M (1996-01-01). "Assessment of biodegradability of plastics under simulated composting conditions in a laboratory test system". International Biodeterioration & Biodegradation. 37 (1–2): 85–92. doi:10.1016/0964-8305(95)00089-5.
- Żenkiewicz M, Malinowski R, Rytlewski P, Richert A, Sikorska W, Krasowska K (2012-02-01). "Some composting and biodegradation effects of physically or chemically crosslinked poly(lactic acid)". Polymer Testing. 31 (1): 83–92. doi:10.1016/j.polymertesting.2011.09.012.
- Avella M, Bonadies E, Martuscelli E, Rimedio R (2001-01-01). "European current standardization for plastic packaging recoverable through composting and biodegradation". Polymer Testing. 20 (5): 517–521. doi:10.1016/S0142-9418(00)00068-4.
- Akullian A, Karp C, Austin K, Durbin D (2006). "Plastic Bag Externalities and Policy in Rhode Island" (PDF). Brown Policy Review. Archived (PDF) from the original on 2017-05-19. Retrieved 2018-09-24.
- Song JH, Murphy RJ, Narayan R, Davies GB (July 2009). "Biodegradable and compostable alternatives to conventional plastics". Philosophical Transactions of the Royal Society of London. Series B, Biological Sciences. 364 (1526): 2127–39. doi:10.1098/rstb.2008.0289. PMC 2873018. PMID 19528060.
- Webb H, Arnott J, Crawford R, Ivanova E, Webb HK, Arnott J, Crawford RJ, Ivanova EP (2012-12-28). "Plastic Degradation and Its Environmental Implications with Special Reference to Poly(ethylene terephthalate)". Polymers. 5 (1): 1–18. doi:10.3390/polym5010001.
- Kelly BC, Ikonomou MG, Blair JD, Morin AE, Gobas FA (July 2007). "Food web-specific biomagnification of persistent organic pollutants". Science. 317 (5835): 236–9. Bibcode:2007Sci...317..236K. doi:10.1126/science.1138275. PMID 17626882. S2CID 52835862.
- Passos CJ, Mergler D (2008). "Human mercury exposure and adverse health effects in the Amazon: a review". Cadernos de Saude Publica. 24 Suppl 4: s503–20. doi:10.1590/s0102-311x2008001600004. PMID 18797727.
- Rana SV (July 2014). "Perspectives in endocrine toxicity of heavy metals--a review". Biological Trace Element Research. 160 (1): 1–14. doi:10.1007/s12011-014-0023-7. PMID 24898714. S2CID 18562345.
- Newman S, Watkins E, Farmer A, Brink Pt, Schweitzer J (2015). "The Economics of Marine Litter". Marine Anthropogenic Litter. Springer International Publishing. pp. 367–394. doi:10.1007/978-3-319-16510-3_14. ISBN 978-3-319-16509-7.
- Matsangou E (2 July 2018). "Counting the cost of plastic pollution". World Finance. Archived from the original on 17 September 2018. Retrieved 17 September 2018.
- Rochman CM, Cook AM, Koelmans AA (July 2016). "Plastic debris and policy: Using current scientific understanding to invoke positive change". Environmental Toxicology and Chemistry. 35 (7): 1617–26. doi:10.1002/etc.3408. PMID 27331654.
- Montanari S (2017-07-25). "Plastic Garbage Patch Bigger Than Mexico Found in Pacific". National Geographic. Archived from the original on 2018-09-17. Retrieved 2018-09-17.
- Gregory MR (July 2009). "Environmental implications of plastic debris in marine settings--entanglement, ingestion, smothering, hangers-on, hitch-hiking and alien invasions". Philosophical Transactions of the Royal Society of London. Series B, Biological Sciences. 364 (1526): 2013–25. doi:10.1098/rstb.2008.0265. PMC 2873013. PMID 19528053.
- Villarrubia-Gómez P, Cornell SE, Fabres J (2018-10-01). "Marine plastic pollution as a planetary boundary threat – The drifting piece in the sustainability puzzle". Marine Policy. 96: 213–220. doi:10.1016/j.marpol.2017.11.035.
- Hajat A, Hsia C, O'Neill MS (December 2015). "Socioeconomic Disparities and Air Pollution Exposure: a Global Review". Current Environmental Health Reports. 2 (4): 440–50. doi:10.1007/s40572-015-0069-5. PMC 4626327. PMID 26381684.
- "Definition of BIODEGRADABLE". www.merriam-webster.com. Archived from the original on 2018-09-24. Retrieved 2018-09-24.
Standards by ASTM International
- D5210- Standard Test Method for Determining the Anaerobic Biodegradation of Plastic Materials in the Presence of Municipal Sewage Sludge
- D5526- Standard Test Method for Determining Anaerobic Biodegradation of Plastic Materials Under Accelerated Landfill Conditions
- D5338- Standard Test Method for Determining Aerobic Biodegradation of Plastic Materials Under Controlled Composting Conditions, Incorporating Thermophilic Temperatures
- D5511- Standard Test Method for Determining Anaerobic Biodegradation of Plastic Materials Under High-Solids Anaerobic-Digestion Conditions
- D5864- Standard Test Method for Determining Aerobic Aquatic Biodegradation of Lubricants or Their Components
- D5988- Standard Test Method for Determining Aerobic Biodegradation of Plastic Materials in Soil
- D6139- Standard Test Method for Determining the Aerobic Aquatic Biodegradation of Lubricants or Their Components Using the Gledhill Shake Flask
- D6006- Standard Guide for Assessing Biodegradability of Hydraulic Fluids
- D6340- Standard Test Methods for Determining Aerobic Biodegradation of Radiolabeled Plastic Materials in an Aqueous or Compost Environment
- D6691- Standard Test Method for Determining Aerobic Biodegradation of Plastic Materials in the Marine Environment by a Defined Microbial Consortium or Natural Sea Water Inoculum
- D6731-Standard Test Method for Determining the Aerobic, Aquatic Biodegradability of Lubricants or Lubricant Components in a Closed Respirometer
- D6954- Standard Guide for Exposing and Testing Plastics that Degrade in the Environment by a Combination of Oxidation and Biodegradation
- D7044- Standard Specification for Biodegradable Fire Resistant Hydraulic Fluids
- D7373-Standard Test Method for Predicting Biodegradability of Lubricants Using a Bio-kinetic Model
- D7475- Standard Test Method for Determining the Aerobic Degradation and Anaerobic Biodegradation of Plastic Materials under Accelerated Bioreactor Landfill Conditions
- D7665- Standard Guide for Evaluation of Biodegradable Heat Transfer Fluids