Striatum
The striatum (PL: striata), or corpus striatum[5] (also called the striate nucleus), is a nucleus (a cluster of neurons) in the subcortical basal ganglia of the forebrain. The striatum is a critical component of the motor and reward systems; receives glutamatergic and dopaminergic inputs from different sources; and serves as the primary input to the rest of the basal ganglia.
Striatum | |
---|---|
![]() | |
![]() Tractography showing corticostriatal connections | |
Details | |
Part of | Basal ganglia[1] Reward system[2][3] |
Parts | Ventral striatum[2][3][4] Dorsal striatum[2][3][4] |
Identifiers | |
Latin | striatum |
MeSH | D003342 |
NeuroNames | 225 |
NeuroLex ID | birnlex_1672 |
TA98 | A14.1.09.516 A14.1.09.515 |
TA2 | 5559 |
FMA | 77616 77618, 77616 |
Anatomical terms of neuroanatomy |
Functionally, the striatum coordinates multiple aspects of cognition, including both motor and action planning, decision-making, motivation, reinforcement, and reward perception.[2][3][4] The striatum is made up of the caudate nucleus and the lentiform nucleus.[6][7] The lentiform nucleus is made up of the larger putamen, and the smaller globus pallidus.[8] Strictly speaking the globus pallidus is part of the striatum. It is common practice, however, to implicitly exclude the globus pallidus when referring to striatal structures.
In primates, the striatum is divided into a ventral striatum, and a dorsal striatum, subdivisions that are based upon function and connections. The ventral striatum consists of the nucleus accumbens and the olfactory tubercle. The dorsal striatum consists of the caudate nucleus and the putamen. A white matter, nerve tract (the internal capsule) in the dorsal striatum separates the caudate nucleus and the putamen.[4] Anatomically, the term striatum describes its striped (striated) appearance of grey-and-white matter.[9]
Structure
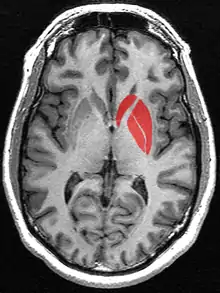
The striatum is the largest structure of the basal ganglia. The striatum is divided into a ventral and a dorsal subdivision, based upon function and connections.
The ventral striatum is composed of the nucleus accumbens and the olfactory tubercle.[4][10] The nucleus accumbens is made up of the nucleus accumbens core and the nucleus accumbens shell, which differ by neural populations. The olfactory tubercle receives input from the olfactory bulb but has not been shown to play a role in processing smell.[10] In non-primate species, the islands of Calleja are included.[11] The ventral striatum is associated with the limbic system and has been implicated as a vital part of the circuitry for decision making and reward-related behavior.[12][13]
The dorsal striatum is composed of the caudate nucleus and the putamen.
Staining can differentiate the striatum into two distinct compartments of striosomes or patches, and a surrounding matrix; this is particularly evident on the components of acetylcholinesterase and calbindin. More studies have been carried out on the dorsal striatum but the compartments have also been identified in the ventral striatum. In the dorsal striatum striosomes make up 10-15 per cent of the striatal volume.[14]
Cell types

Types of cells in the striatum include:
- Medium spiny neurons (MSNs), which are the principal neurons of the striatum.[2] They are GABAergic and, thus, are classified as inhibitory neurons. Medium spiny projection neurons comprise 95% of the total neuronal population of the human striatum.[2] Medium spiny neurons have two characteristic types: D1-type MSNs and D2-type MSNs.[2][4][15] A subpopulation of MSNs contain both D1-type and D2-type receptors, with approximately 40% of striatal MSNs expressing both DRD1 and DRD2 mRNA.[2][4][15]
- Cholinergic interneurons release acetylcholine, which has a variety of important effects in the striatum. In humans, other primates, and rodents, these interneurons respond to salient environmental stimuli with stereotyped responses that are temporally aligned with the responses of dopaminergic neurons of the substantia nigra.[16][17] The large aspiny cholinergic interneurons themselves are affected by dopamine through D5 dopamine receptors.[18] Dopamine also directly controls communication between cholinergic interneurons.[19][20]
- There are many types of GABAergic interneurons.[21] The best known are parvalbumin expressing interneurons, also known as fast-spiking interneurons, which participate in powerful feedforward inhibition of principal neurons.[22] Also, there are GABAergic interneurons that express tyrosine hydroxylase,[23] somatostatin, nitric oxide synthase and neuropeptide-y. Recently, two types of neuropeptide-y expressing GABAergic interneurons have been described in detail,[24] one of which translates synchronous activity of cholinergic interneurons into inhibition of principal neurons.[25] These neurons of the striatum are not distributed evenly.[21]
There are two regions of neurogenesis in the brain – the subventricular zone in the lateral ventricles, and the dentate gyrus in the hippocampal formation. Neuroblasts that form in the lateral ventricle adjacent to the striatum, integrate in the striatum.[26][27] This has been noted in the human striatum following an ischemic stroke. Injury caused to the striatum stimulates the migration of neuroblasts from the subventricular zone, to the striatum, where they differentiate into adult neurons.[28] The normal passage of SVZ neuroblasts is to the olfactory bulb but this traffic is diverted to the striatum after an ischemic stroke. However, few of the new developed neurons survive.[29]
Inputs


[30] The largest connection is from the cortex, in terms of cell axons. Many parts of the neocortex innervate the dorsal striatum. The cortical pyramidal neurons projecting to the striatum are located in layers II-VI, with the most dense projections come from layer V.[31] They end mainly on the dendritic spines of the spiny neurons. They are glutamatergic, exciting striatal neurons.
The striatum is seen as having its own internal microcircuitry.[32] The ventral striatum receives direct input from multiple regions in the cerebral cortex and limbic structures such as the amygdala, thalamus, and hippocampus, as well as the entorhinal cortex and the inferior temporal gyrus.[33] Its primary input is to the basal ganglia system. Additionally, the mesolimbic pathway projects from the ventral tegmental area to the nucleus accumbens of the ventral striatum.[34]
Another well-known afferent is the nigrostriatal connection arising from the neurons of the substantia nigra pars compacta. While cortical axons synapse mainly on spine heads of spiny neurons, nigral axons synapse mainly on spine shafts. In primates, the thalamostriatal afferent comes from the central median-parafascicular complex of the thalamus (see primate basal ganglia system). This afferent is glutamatergic. The participation of truly intralaminar neurons is much more limited. The striatum also receives afferents from other elements of the basal ganglia such as the subthalamic nucleus (glutamatergic) or the external globus pallidus (GABAergic).
Targets
The primary outputs of the ventral striatum project to the ventral pallidum, then the medial dorsal nucleus of the thalamus, which is part of the frontostriatal circuit. Additionally, the ventral striatum projects to the globus pallidus, and substantia nigra pars reticulata. Some of its other outputs include projections to the extended amygdala, lateral hypothalamus, and pedunculopontine nucleus.[35]
Striatal outputs from both the dorsal and ventral components are primarily composed of medium spiny neurons (MSNs), a type of projection neuron, which have two primary phenotypes: "indirect" MSNs that express D2-like receptors and "direct" MSNs that express D1-like receptors.[2][4]
The main nucleus of the basal ganglia is the striatum which projects directly to the globus pallidus via a pathway of striatopallidal fibers.[36] The striato-pallidal pathway has a whitish appearance due to the myelinated fibers. This projection comprises successively the external globus pallidus (GPe), the internal globus pallidus (GPi), the pars compacta of the substantia nigra (SNc), and the pars reticulata of substantia nigra (SNr). The neurons of this projection are inhibited by GABAergic synapses from the dorsal striatum. Among these targets, the GPe does not send axons outside the system. Others send axons to the superior colliculus. Two others comprise the output to the thalamus, forming two separate channels: one through the internal segment of the globus pallidus to the ventral oralis nuclei of the thalamus and from there to the cortical supplementary motor area and another through the substantia nigra to the ventral anterior nuclei of the thalamus and from there to the frontal cortex and the occulomotor cortex.
Blood supply
Deep penetrating striate arteries supply blood to the striatum. These arteries include the recurrent artery of Heubner arising from the anterior cerebral artery, and the lenticulostriate arteries arising from the middle cerebral artery.[37]
Function
The ventral striatum, and the nucleus accumbens in particular, primarily mediates reward, cognition, reinforcement, and motivational salience, whereas the dorsal striatum primarily mediates cognition involving motor function, certain executive functions (e.g., inhibitory control and impulsivity), and stimulus-response learning;[2][3][4][38][39] there is a small degree of overlap, as the dorsal striatum is also a component of the reward system that, along with the nucleus accumbens core, mediates the encoding of new motor programs associated with future reward acquisition (e.g., the conditioned motor response to a reward cue).[3][38]
Metabotropic dopamine receptors are present both on spiny neurons and on cortical axon terminals. Second messenger cascades triggered by activation of these dopamine receptors can modulate pre- and postsynaptic function, both in the short term and in the long term.[40][41] In humans, the striatum is activated by stimuli associated with reward, but also by aversive, novel,[42] unexpected, or intense stimuli, and cues associated with such events.[43] fMRI evidence suggests that the common property linking these stimuli, to which the striatum is reacting, is salience under the conditions of presentation.[44][45] A number of other brain areas and circuits are also related to reward, such as frontal areas. Functional maps of the striatum reveal interactions with widely distributed regions of the cerebral cortex important to a diverse range of functions.[46]
The interplay between the striatum and the prefrontal cortex is relevant for behavior, particularly adolescent development as proposed by the dual systems model.[47]
Clinical significance
Parkinson's disease and other movement disorders
Parkinson's disease results in loss of dopaminergic innervation to the dorsal striatum (and other basal ganglia) and a cascade of consequences. Atrophy of the striatum is also involved in Huntington's disease, and movement disorders such as chorea, choreoathetosis, and dyskinesias.[48] These have also been described as circuit disorders of the basal ganglia.[49]
Addiction
Addiction, a disorder of the brain's reward system, arises through the overexpression of DeltaFosB (ΔFosB), a transcription factor, in the D1-type medium spiny neurons of the ventral striatum. ΔFosB is an inducible gene which is increasingly expressed in the nucleus accumbens as a result of repeatedly using an addictive drug or overexposure to other addictive stimuli.[50][51]
Bipolar disorder
An association has been observed between striatal expression of variants of the PDE10A gene and some bipolar I disorder patients. Variants of other genes, DISC1 and GNAS, have been associated with bipolar II disorder.[52]
Autism spectrum disorder
Autism spectrum disorder (ASD) is characterized by cognitive inflexibility and poor understanding of social systems. This inflexible behavior originates in defects in the pre-frontal cortex as well as the striatal circuits.[53] The defects in the striatum seem to specifically contribute to the motor, social and communication impairments seen in ASD patients. In mice which have an ASD-like phenotype induced via the overexpression of the eukaryotic initiation of translation factor 4E, it has been shown that these defects seem to stem from the reduced ability to store and process information in the striatum, which leads to the difficulty seen in forming new motor patterns, as well as disengaging from existing ones.[54]
Dysfunction
Dysfunction in the ventral striatum can lead to a variety of disorders, most notably, depression and obsessive-compulsive disorder. Because of its involvement in reward pathways, the ventral striatum has also been implicated in playing a critical role in addiction. It has been well established that the ventral striatum is strongly involved in mediating the reinforcing effects of drugs, especially stimulants, through dopaminergic stimulation.[55]
History
In the seventeenth and eighteenth centuries, the term "corpus striatum" was used to designate many distinct, deep, infracortical elements of the hemisphere.[56] Etymologically it is derived from (Latin) "striatus" [57] = "grooved, striated" and the English "striated" = having parallel lines or grooves on the surface.[58] In 1876 David Ferrier contributed decades of research to the subject; concluding that the corpus striatum was vital in the "organization and generation of voluntary movement".[59][60][61][62][63] In 1941, Cécile and Oskar Vogt simplified the nomenclature by proposing the term striatum for all elements in the basal ganglia built with striatal elements: the caudate nucleus, the putamen, and the fundus striati,[64] which is the ventral part linking the two preceding together ventrally to the inferior part of the internal capsule.
The term neostriatum was forged by comparative anatomists comparing the subcortical structures between vertebrates, because it was thought to be a phylogenetically newer section of the corpus striatum. The term is still used by some sources, including Medical Subject Headings.[65]
Other animals
In birds the term used was the paleostriatum augmentatum, and in the new avian terminology listing (as of 2002) for neostriatum this has been changed to the nidopallium.[66]
In non-primate species, the islands of Calleja are included in the ventral striatum.[11]
See also
Additional images
- Striatum highlighted in green on coronal T1 MRI images
- Striatum highlighted in green on sagittal T1 MRI images
- Striatum highlighted in green on transversal T1 MRI images
References
- "Basal ganglia". BrainInfo. Retrieved 16 August 2015.
- Yager LM, Garcia AF, Wunsch AM, Ferguson SM (August 2015). "The ins and outs of the striatum: Role in drug addiction". Neuroscience. 301: 529–541. doi:10.1016/j.neuroscience.2015.06.033. PMC 4523218. PMID 26116518.
[The striatum] receives dopaminergic inputs from the ventral tegmental area (VTA) and the substantia nigra (SNr) and glutamatergic inputs from several areas, including the cortex, hippocampus, amygdala, and thalamus (Swanson, 1982; Phillipson and Griffiths, 1985; Finch, 1996; Groenewegen et al., 1999; Britt et al., 2012). These glutamatergic inputs make contact on the heads of dendritic spines of the striatal GABAergic medium spiny projection neurons (MSNs) whereas dopaminergic inputs synapse onto the spine neck, allowing for an important and complex interaction between these two inputs in modulation of MSN activity ... It should also be noted that there is a small population of neurons in the NAc that coexpress both D1 and D2 receptors, though this is largely restricted to the NAc shell (Bertran- Gonzalez et al., 2008). ... Neurons in the NAc core and NAc shell subdivisions also differ functionally. The NAc core is involved in the processing of conditioned stimuli whereas the NAc shell is more important in the processing of unconditioned stimuli; Classically, these two striatal MSN populations are thought to have opposing effects on basal ganglia output. Activation of the dMSNs causes a net excitation of the thalamus resulting in a positive cortical feedback loop; thereby acting as a 'go' signal to initiate behavior. Activation of the iMSNs, however, causes a net inhibition of thalamic activity resulting in a negative cortical feedback loop and therefore serves as a 'brake' to inhibit behavior ... there is also mounting evidence that iMSNs play a role in motivation and addiction (Lobo and Nestler, 2011; Grueter et al., 2013). ... Together these data suggest that iMSNs normally act to restrain drug-taking behavior and recruitment of these neurons may in fact be protective against the development of compulsive drug use.
- Taylor SB, Lewis CR, Olive MF (February 2013). "The neurocircuitry of illicit psychostimulant addiction: acute and chronic effects in humans". Subst. Abuse Rehabil. 4: 29–43. doi:10.2147/SAR.S39684. PMC 3931688. PMID 24648786.
The DS (also referred to as the caudate-putamen in primates) is associated with transitions from goal-directed to habitual drug use, due in part to its role in stimulus–response learning.28,46 As described above, the initial rewarding and reinforcing effects of drugs of abuse are mediated by increases in extracellular DA in the NAc shell, and after continued drug use in the NAc core.47,48 After prolonged drug use, drug-associated cues produce increases in extracellular DA levels in the DS and not in the NAc.49 This lends to the notion that a shift in the relative engagement from the ventral to the dorsal striatum underlies the progression from initial, voluntary drug use to habitual and compulsive drug use.28 In addition to DA, recent evidence indicates that glutamatergic transmission in the DS is important for drug-induced adaptations and plasticity within the DS.50
- Ferré S, Lluís C, Justinova Z, Quiroz C, Orru M, Navarro G, Canela EI, Franco R, Goldberg SR (June 2010). "Adenosine-cannabinoid receptor interactions. Implications for striatal function". Br. J. Pharmacol. 160 (3): 443–453. doi:10.1111/j.1476-5381.2010.00723.x. PMC 2931547. PMID 20590556.
Two classes of MSNs, which are homogeneously distributed in the striatum, can be differentiated by their output connectivity and their expression of dopamine and adenosine receptors and neuropeptides. In the dorsal striatum (mostly represented by the nucleus caudate-putamen), enkephalinergic MSNs connect the striatum with the external globus pallidus and express the peptide enkephalin and a high density of dopamine D2 and adenosine A2A receptors (they also express adenosine A1 receptors), while dynorphinergic MSNs connect the striatum with the substantia nigra (pars compacta and reticulata) and the entopeduncular nucleus (internal globus pallidus) and express the peptides dynorphin and substance P and dopamine D1 and adenosine A1 but not A2A receptors ... These two different phenotypes of MSN are also present in the ventral striatum (mostly represented by the nucleus accumbens and the olfactory tubercle). However, although they are phenotypically equal to their dorsal counterparts, they have some differences in terms of connectivity. First, not only enkephalinergic but also dynorphinergic MSNs project to the ventral counterpart of the external globus pallidus, the ventral pallidum, which, in fact, has characteristics of both the external and internal globus pallidus in its afferent and efferent connectivity. In addition to the ventral pallidum, the internal globus pallidus and the substantia nigra-VTA, the ventral striatum sends projections to the extended amygdala, the lateral hypothalamus and the pedunculopontine tegmental nucleus. ... It is also important to mention that a small percentage of MSNs have a mixed phenotype and express both D1 and D2 receptors (Surmeier et al., 1996).
- "striatum | Definition of striatum in English by Oxford Dictionaries". Oxford Dictionaries | English. Archived from the original on 18 January 2018. Retrieved 17 January 2018.
- Jones, Jeremy. "Corpus striatum | Radiology Reference Article | Radiopaedia.org". radiopaedia.org. Retrieved 17 January 2018.
- "Corpus striatum". BrainInfo. Retrieved 16 August 2015.
- Telford, Ryan; Vattoth, Surjith (February 2014). "MR Anatomy of Deep Brain Nuclei with Special Reference to Specific Diseases and Deep Brain Stimulation Localization". The Neuroradiology Journal. 27 (1): 29–43. doi:10.15274/NRJ-2014-10004. PMC 4202840. PMID 24571832.
- "Striatum definition and meaning | Collins English Dictionary". www.collinsdictionary.com.
- Ubeda-Bañon I, Novejarque A, Mohedano-Moriano A, et al. (2007). "Projections from the posterolateral olfactory amygdala to the ventral striatum: neural basis for reinforcing properties of chemical stimuli". BMC Neurosci. 8: 103. doi:10.1186/1471-2202-8-103. PMC 2216080. PMID 18047654.
- "Ventral striatum – NeuroLex". neurolex.org. Retrieved 12 December 2015.
- "Ventral Striatum Definition – Medical Dictionary". medicaldictionary.net. Retrieved 18 November 2015.
- "Ventral Striatum – Medical Definition". www.medilexicon.com. Retrieved 18 November 2015.
- Brimblecombe, K. R.; Cragg, S. J. (2017). "The Striosome and Matrix Compartments of the Striatum: A Path through the Labyrinth from Neurochemistry toward Function". ACS Chemical Neuroscience. 8 (2): 235–242. doi:10.1021/acschemneuro.6b00333. PMID 27977131.
- Nishi, Akinori; Kuroiwa, Mahomi; Shuto, Takahide (2011). "Mechanisms for the Modulation of Dopamine D1 Receptor Signaling in Striatal Neurons". Frontiers in Neuroanatomy. 5: 43. doi:10.3389/fnana.2011.00043. PMC 3140648. PMID 21811441.
- Goldberg, J.A.; Reynolds, J.N.J. (December 2011). "Spontaneous firing and evoked pauses in the tonically active cholinergic interneurons of the striatum". Neuroscience. 198: 27–43. doi:10.1016/j.neuroscience.2011.08.067. PMID 21925242. S2CID 21908514.
- Morris, Genela; Arkadir, David; Nevet, Alon; Vaadia, Eilon; Bergman, Hagai (July 2004). "Coincident but Distinct Messages of Midbrain Dopamine and Striatal Tonically Active Neurons". Neuron. 43 (1): 133–143. doi:10.1016/j.neuron.2004.06.012. PMID 15233923.
- Bergson, C; Mrzljak, L; Smiley, JF; Pappy, M; Levenson, R; Goldman-Rakic, PS (1 December 1995). "Regional, cellular, and subcellular variations in the distribution of D1 and D5 dopamine receptors in primate brain". The Journal of Neuroscience. 15 (12): 7821–7836. doi:10.1523/JNEUROSCI.15-12-07821.1995. PMC 6577925. PMID 8613722.
- Raz, Aeyal (1996). "Neuronal synchronization of tonically active neurons in the striatum of normal and parkinsonian primates". Journal of Neurophysiology. 76 (3): 2083–2088. doi:10.1152/jn.1996.76.3.2083. PMID 8890317.
- Dorst, Matthijs (2020). "Polysynaptic inhibition between striatal cholinergic interneurons shapes their network activity patterns in a dopamine-dependent manner". Nature Communications. 11 (1): 5113. Bibcode:2020NatCo..11.5113D. doi:10.1038/s41467-020-18882-y. PMC 7547109. PMID 33037215.
- Tepper, James M.; Tecuapetla, Fatuel; Koós, Tibor; Ibáñez-Sandoval, Osvaldo (2010). "Heterogeneity and Diversity of Striatal GABAergic Interneurons". Frontiers in Neuroanatomy. 4: 150. doi:10.3389/fnana.2010.00150. PMC 3016690. PMID 21228905.
- Koós, Tibor; Tepper, James M. (May 1999). "Inhibitory control of neostriatal projection neurons by GABAergic interneurons". Nature Neuroscience. 2 (5): 467–472. doi:10.1038/8138. PMID 10321252. S2CID 16088859.
- Ibanez-Sandoval, O.; Tecuapetla, F.; Unal, B.; Shah, F.; Koos, T.; Tepper, J. M. (19 May 2010). "Electrophysiological and Morphological Characteristics and Synaptic Connectivity of Tyrosine Hydroxylase-Expressing Neurons in Adult Mouse Striatum". Journal of Neuroscience. 30 (20): 6999–7016. doi:10.1523/JNEUROSCI.5996-09.2010. PMC 4447206. PMID 20484642.
- Ibanez-Sandoval, O.; Tecuapetla, F.; Unal, B.; Shah, F.; Koos, T.; Tepper, J. M. (16 November 2011). "A Novel Functionally Distinct Subtype of Striatal Neuropeptide Y Interneuron". Journal of Neuroscience. 31 (46): 16757–16769. doi:10.1523/JNEUROSCI.2628-11.2011. PMC 3236391. PMID 22090502.
- English, Daniel F; Ibanez-Sandoval, Osvaldo; Stark, Eran; Tecuapetla, Fatuel; Buzsáki, György; Deisseroth, Karl; Tepper, James M; Koos, Tibor (11 December 2011). "GABAergic circuits mediate the reinforcement-related signals of striatal cholinergic interneurons". Nature Neuroscience. 15 (1): 123–130. doi:10.1038/nn.2984. PMC 3245803. PMID 22158514.
- Ernst, Aurélie; Alkass, Kanar; Bernard, Samuel; Salehpour, Mehran; Perl, Shira; Tisdale, John; Possnert, Göran; Druid, Henrik; Frisén, Jonas (February 2014). "Neurogenesis in the Striatum of the Adult Human Brain". Cell. 156 (5): 1072–1083. doi:10.1016/j.cell.2014.01.044. PMID 24561062.
- Inta, D; Lang, U E; Borgwardt, S; Meyer-Lindenberg, A; Gass, P (16 February 2016). "Adult neurogenesis in the human striatum: possible implications for psychiatric disorders". Molecular Psychiatry. 21 (4): 446–447. doi:10.1038/mp.2016.8. PMID 26878892.
- Kernie, SG; Parent, JM (February 2010). "Forebrain neurogenesis after focal Ischemic and traumatic brain injury". Neurobiology of Disease. 37 (2): 267–74. doi:10.1016/j.nbd.2009.11.002. PMC 2864918. PMID 19909815.
- Yamashita, T; Ninomiya, M; Hernández Acosta, P; García-Verdugo, JM; Sunabori, T; Sakaguchi, M; Adachi, K; Kojima, T; Hirota, Y; Kawase, T; Araki, N; Abe, K; Okano, H; Sawamoto, K (14 June 2006). "Subventricular zone-derived neuroblasts migrate and differentiate into mature neurons in the post-stroke adult striatum" (PDF). The Journal of Neuroscience. 26 (24): 6627–36. doi:10.1523/jneurosci.0149-06.2006. PMC 6674034. PMID 16775151. Archived (PDF) from the original on 9 October 2022.
- Beckstead, Robert M.; Domesick, Valerie B.; Nauta, Walle J.H. (October 1979). "Efferent connections of the substantia nigra and ventral tegmental area in the rat". Brain Research. 175 (2): 191–217. doi:10.1016/0006-8993(79)91001-1. PMID 314832. S2CID 24210605.
- Rosell, Antonio; Giménez-Amaya, José Manuel (September 1999). "Anatomical re-evaluation of the corticostriatal projections to the caudate nucleus: a retrograde labeling study in the cat". Neuroscience Research. 34 (4): 257–269. doi:10.1016/S0168-0102(99)00060-7. PMID 10576548. S2CID 31392396.
- Stocco, Andrea; Lebiere, Christian; Anderson, John R. (2010). "Conditional Routing of Information to the Cortex: A Model of the Basal Ganglia's Role in Cognitive Coordination". Psychological Review. 117 (2): 541–74. doi:10.1037/a0019077. PMC 3064519. PMID 20438237.
- "Ventral striatum – NeuroLex". neurolex.org. Retrieved 12 December 2015.
- "Icahn School of Medicine | Neuroscience Department | Nestler Lab | Brain Reward Pathways". neuroscience.mssm.edu. Retrieved 12 December 2015.
- Robbins, Trevor W.; Everitt, Barry J. (April 1992). "Functions of dopamine in the dorsal and ventral striatum". Seminars in Neuroscience. 4 (2): 119–127. doi:10.1016/1044-5765(92)90010-Y.
- Pujol, S.; Cabeen, R.; Sébille, S. B.; Yelnik, J.; François, C.; Fernandez Vidal, S.; Karachi, C.; Zhao, Y.; Cosgrove, G. R.; Jannin, P.; Kikinis, R.; Bardinet, E. (2016). "In vivo Exploration of the Connectivity between the Subthalamic Nucleus and the Globus Pallidus in the Human Brain Using Multi-Fiber Tractography". Frontiers in Neuroanatomy. 10: 119. doi:10.3389/fnana.2016.00119. PMC 5243825. PMID 28154527.
- Purves, Dale (2012). Neuroscience (5th ed.). Sunderland, Mass. p. 739. ISBN 9780878936953.
{{cite book}}
: CS1 maint: location missing publisher (link) - Malenka RC, Nestler EJ, Hyman SE (2009). Sydor A, Brown RY (eds.). Molecular Neuropharmacology: A Foundation for Clinical Neuroscience (2nd ed.). New York: McGraw-Hill Medical. pp. 147–148, 321, 367, 376. ISBN 978-0-07-148127-4.
VTA DA neurons play a critical role in motivation, reward-related behavior (Chapter 15), attention, and multiple forms of memory. This organization of the DA system, wide projection from a limited number of cell bodies, permits coordinated responses to potent new rewards. Thus, acting in diverse terminal fields, dopamine confers motivational salience ("wanting") on the reward itself or associated cues (nucleus accumbens shell region), updates the value placed on different goals in light of this new experience (orbital prefrontal cortex), helps consolidate multiple forms of memory (amygdala and hippocampus), and encodes new motor programs that will facilitate obtaining this reward in the future (nucleus accumbens core region and dorsal striatum). In this example, dopamine modulates the processing of sensorimotor information in diverse neural circuits to maximize the ability of the organism to obtain future rewards. ...
Functional neuroimaging in humans demonstrates activation of the prefrontal cortex and caudate nucleus (part of the striatum) in tasks that demand inhibitory control of behavior. ...
The brain reward circuitry that is targeted by addictive drugs normally mediates the pleasure and strengthening of behaviors associated with natural reinforcers, such as food, water, and sexual contact. Dopamine neurons in the VTA are activated by food and water, and dopamine release in the NAc is stimulated by the presence of natural reinforcers, such as food, water, or a sexual partner. ...
The NAc and VTA are central components of the circuitry underlying reward and memory of reward. As previously mentioned, the activity of dopaminergic neurons in the VTA appears to be linked to reward prediction. The NAc is involved in learning associated with reinforcement and the modulation of motoric responses to stimuli that satisfy internal homeostatic needs. The shell of the NAc appears to be particularly important to initial drug actions within reward circuitry; addictive drugs appear to have a greater effect on dopamine release in the shell than in the core of the NAc. - Kim, BaekSun; Im, Heh-In (2019). "The role of the dorsal striatum in choice impulsivity". Annals of the New York Academy of Sciences. 1451 (1): 92–111. Bibcode:2019NYASA1451...92K. doi:10.1111/nyas.13961. PMID 30277562. S2CID 52897511.
- Greengard, P (2001). "The neurobiology of slow synaptic transmission". Science. 294 (5544): 1024–30. Bibcode:2001Sci...294.1024G. doi:10.1126/science.294.5544.1024. PMID 11691979.
- Cachope, R; Cheer (2014). "Local control of striatal dopamine release". Frontiers in Behavioral Neuroscience. 8: 188. doi:10.3389/fnbeh.2014.00188. PMC 4033078. PMID 24904339.
- UCL (25 June 2008). "Adventure - it's all in the mind, say UCL neuroscientists". UCL News.
- Volman, S. F.; Lammel; Margolis; Kim; Richard; Roitman; Lobo (2013). "New insights into the specificity and plasticity of reward and aversion encoding in the mesolimbic system". Journal of Neuroscience. 33 (45): 17569–76. doi:10.1523/JNEUROSCI.3250-13.2013. PMC 3818538. PMID 24198347.
- LUNA, BEATRIZ; SWEENEY, JOHN A. (1 June 2004). "The Emergence of Collaborative Brain Function: fMRI Studies of the Development of Response Inhibition". Annals of the New York Academy of Sciences. 1021 (1): 296–309. Bibcode:2004NYASA1021..296L. doi:10.1196/annals.1308.035. PMID 15251900. S2CID 37404147.
- "Department of Physiology, Development and Neuroscience: About the Department".
- Choi EY, Yeo BT, Buckner RL (2012). "The organization of the human striatum estimated by intrinsic functional connectivity". Journal of Neurophysiology. 108 (8): 2242–2263. doi:10.1152/jn.00270.2012. PMC 3545026. PMID 22832566.
- Steinberg, Laurence (April 2010). "A dual systems model of adolescent risk-taking". Developmental Psychobiology. 52 (3): 216–224. doi:10.1002/dev.20445. ISSN 1098-2302. PMID 20213754.
- Walker FO (January 2007). "Huntington's disease". Lancet. 369 (9557): 218–28. doi:10.1016/S0140-6736(07)60111-1. PMID 17240289. S2CID 46151626.
- Delong, M. R.; Wichmann, T. (2007). "Circuits and circuit disorders of the basal ganglia". Archives of Neurology. 64 (1): 20–4. doi:10.1001/archneur.64.1.20. PMID 17210805.
- Nestler EJ (December 2013). "Cellular basis of memory for addiction". Dialogues Clin. Neurosci. 15 (4): 431–443. doi:10.31887/DCNS.2013.15.4/enestler. PMC 3898681. PMID 24459410.
- Olsen CM (December 2011). "Natural rewards, neuroplasticity, and non-drug addictions". Neuropharmacology. 61 (7): 1109–22. doi:10.1016/j.neuropharm.2011.03.010. PMC 3139704. PMID 21459101.
Table 1 - McDonald, M-L; MacMullen, C; Liu, D J; Leal, S M; Davis, R L (2 October 2012). "Genetic association of cyclic AMP signaling genes with bipolar disorder". Translational Psychiatry. 2 (10): e169. doi:10.1038/tp.2012.92. PMC 3565822. PMID 23032945.
- Fineberg, Naomi A; Potenza, Marc N; Chamberlain, Samuel R; Berlin, Heather A; Menzies, Lara; Bechara, Antoine; Sahakian, Barbara J; Robbins, Trevor W; Bullmore, Edward T; Hollander, Eric (25 November 2009). "Probing Compulsive and Impulsive Behaviors, from Animal Models to Endophenotypes: A Narrative Review". Neuropsychopharmacology. 35 (3): 591–604. doi:10.1038/npp.2009.185. PMC 3055606. PMID 19940844.
- Santini, Emanuela; Huynh, Thu N.; MacAskill, Andrew F.; Carter, Adam G.; Pierre, Philippe; Ruggero, Davide; Kaphzan, Hanoch; Klann, Eric (23 December 2012). "Exaggerated translation causes synaptic and behavioural aberrations associated with autism". Nature. 493 (7432): 411–415. Bibcode:2013Natur.493..411S. doi:10.1038/nature11782. PMC 3548017. PMID 23263185.
- Everitt, Barry J.; Robbins, Trevor W. (November 2013). "From the ventral to the dorsal striatum: Devolving views of their roles in drug addiction". Neuroscience & Biobehavioral Reviews. 37 (9): 1946–1954. doi:10.1016/j.neubiorev.2013.02.010. PMID 23438892.
- Raymond Vieussens, 1685
- "Striatus". 16 August 2019.
- "Striated". 9 November 2019.
- Hikosaka, O. (1998). "Neural systems for control of voluntary action--a hypothesis". Advances in Biophysics. 35: 81–102. doi:10.1016/S0065-227X(98)80004-X. ISSN 0065-227X. PMID 9949766.
- Graybiel, Ann M.; Aosaki, Toshihiko; Flaherty, Alice W.; Kimura, Minoru (23 September 1994). "The Basal Ganglia and Adaptive Motor Control". Science. 265 (5180): 1826–1831. Bibcode:1994Sci...265.1826G. doi:10.1126/science.8091209. ISSN 0036-8075. PMID 8091209.
- Bromberg-Martin, Ethan S.; Matsumoto, Masayuki; Hikosaka, Okihide (9 December 2010). "Dopamine in Motivational Control: Rewarding, Aversive, and Alerting". Neuron. 68 (5): 815–834. doi:10.1016/j.neuron.2010.11.022. ISSN 0896-6273. PMC 3032992. PMID 21144997.
- Ferrier, David (1 July 1877). "Ferrier on the Functions of the Brain". The British and Foreign Medico-Chirurgical Review. 60 (119): 99–114. PMC 5199255. PMID 30164726.
- Kravitz, Alexxai V.; Kreitzer, Anatol C. (1 June 2012). "Striatal Mechanisms Underlying Movement, Reinforcement, and Punishment". Physiology. 27 (3): 167–177. doi:10.1152/physiol.00004.2012. ISSN 1548-9213. PMC 3880226. PMID 22689792.
- "NeuroNames Ancillary: fundus striati". braininfo.rprc.washington.edu. Retrieved 17 January 2018.
- Neostriatum at the U.S. National Library of Medicine Medical Subject Headings (MeSH)
- "New Terminology for the Neostriatum". www.avianbrain.org. Retrieved 17 January 2018.
External links

- Stained brain slice images which include the "striatum" at the BrainMaps project
- hier-207 at NeuroNames
- Corpus+Striatum at the U.S. National Library of Medicine Medical Subject Headings (MeSH)
- https://web.archive.org/web/20131029195257/http://www.nimh.nih.gov/images/news-items/r1_braindorsal1.jpg
- https://web.archive.org/web/20090914200329/http://www.hnl.bcm.tmc.edu/fmri.html