Tipping points in the climate system
In climate science, a tipping point is a critical threshold that, when crossed, leads to large and often irreversible changes in the climate system.[1] If tipping points are crossed, they are likely to have severe impacts on human society.[2][3] Tipping behaviour is found across the climate system, in ecosystems, ice sheets, and the circulation of the ocean and atmosphere.[3]
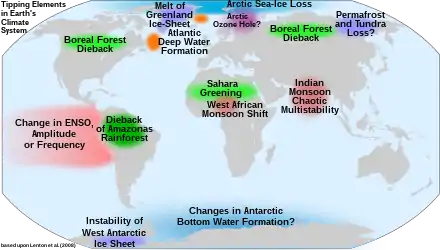
Tipping points are often, but not necessarily, abrupt. For example, with average global warming somewhere between 0.8 and 3 °C, the Greenland ice sheet passes a tipping point and is doomed, but the melt would take place over millennia.[4][5]
Tipping points are possible at today's global warming of just over 1 °C above preindustrial times, and highly probable above 2 °C of global warming.[3] The geological record shows many abrupt changes that suggest tipping points may have been crossed in ancient times.[6] It is possible that some tipping points are close to being crossed or have already been crossed, like those of the West Antarctic and Greenland ice sheets, the Amazon rainforest and warm-water coral reefs.[7] A danger is that if the tipping point in one system is crossed, this could cause a cascade of other tipping points, leading to severe impacts.[8]
Definition
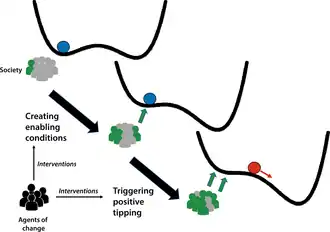
The sixth report from the United Nations Intergovernmental Panel on Climate Change (IPCC), released in 2021, defines a tipping point as a "critical threshold beyond which a system reorganizes, often abruptly and/or irreversibly".[9] It can be brought about by a small disturbance causing a disproportionately large change in the system. One set of definitions of "tipping points" also requires self-reinforcing feedbacks, which could lead to changes in the climate system irreversible on a human timescale.[10] For any particular climate component, the shift from one state to a new stable state may take many decades or centuries.[10] In ecosystems and in social systems, a tipping point can trigger a regime shift, a major systems reorganisation into a new stable state.[11]
The 2019 IPCC Special Report on the Ocean and Cryosphere in a Changing Climate defines a tipping point as: "A level of change in system properties beyond which a system reorganises, often in a non-linear manner, and does not return to the initial state even if the drivers of the change are abated. For the climate system, the term refers to a critical threshold at which global or regional climate changes from one stable state to another stable state.".[12]
Geological record

The geological record shows that there have been abrupt changes in the climate system that indicate ancient tipping points. For instance, the Dansgaard–Oeschger events during the last ice age were periods of abrupt warming (within decades) in Greenland and Europe, that may have involved the abrupt changes in major ocean currents. During the deglaciation in the early Holocene, sea level rise was not smooth, but rose abruptly during meltwater pulses. The monsoon in North Africa saw abrupt changes on decadal timescales during the African humid period. This period, spanning from 15,000 to 5,000 years ago, also ended suddenly in a drier state.[6]
Tipping elements
Scientists have identified many elements in the climate system which may have tipping points.[13][10] in the early 2000s the IPCC began considering the possibility of tipping points. At that time the IPCC concluded they would only be likely in the event of global warming of 4 °C or more above preindustrial times. As of 2021 tipping points are considered to have significant probability at today's warming level of just over 1 °C, with high probability above 2 °C of global warming,[3] and it is possible that some tipping points are close to being crossed or have already been crossed, like those of the ice sheets in West Antarctic and Greenland, warm-water coral reefs, and the Amazon rainforest.[14][15] As of September 2022, nine core tipping elements and seven regional impact tipping elements were identified. Out of those, one regional and three core climate elements are estimated to likely pass a tipping point if global warming reaches 1.5 °C, namely the Greenland ice sheet collapse, the West Antarctic ice sheet collapse, tropical coral reef die off, and the boreal permafrost abrupt thaw. Two further tipping points are forecast as likely if warming continues to approach 2 °C : Barents sea ice abrupt loss, and the Labrador sea subpolar gyre collapse.[4][16][5]
Proposed climate tipping element (and tipping point) | Threshold ( °C) | Timescale (years) | Maximum Impact ( °C) | ||||||
---|---|---|---|---|---|---|---|---|---|
Estimated | Minimum | Maximum | Estimated | Minimum | Maximum | Global | Regional | ||
Greenland Ice Sheet (collapse) | 1.5 | 0.8 | 3.0 | 10k | 1k | 15k | 0.13 | 0.5 to 3.0 | |
West Antarctic Ice Sheet (collapse) | 1.5 | 1.0 | 3.0 | 2k | 500 | 13k | 0.05 | 1.0 | |
Labrador-Irminger Seas/SPG Convection (collapse) | 1.8 | 1.1 | 3.8 | 10 | 5 | 50 | -0.5 | -3.0 | |
East Antarctic Subglacial Basins (collapse) | 3.0 | 2.0 | 6.0 | 2k | 500 | 10k | 0.05 | ? | |
Amazon Rainforest (dieback) | 3.5 | 2.0 | 6.0 | 100 | 50 | 200 | 0.1 (partial) 0.2 (total)[T1 1] | 0.4 to 2.0 | |
Boreal Permafrost (collapse) | 4.0 | 3.0 | 6.0 | 50 | 10 | 300 | 0.2 - 0.4[T1 2] | ~ | |
Atlantic Meridional Overturning Circulation (collapse) | 4 | 1.4 | 8 | 50 | 15 | 300 | -0.5 | -4 to -10 | |
Arctic Winter Sea Ice (collapse) | 6.3 | 4.5 | 8.7 | 20 | 10 | 100 | 0.6 | 0.6 to 1.2 | |
East Antarctic Ice Sheet (collapse) | 7.5 | 5.0 | 10.0 | ? | 10k | ? | 0.6 | 2.0 | |
Low-latitude Coral Reefs (dieoff) | 1.5 | 1.0 | 2.0 | 10 | ~ | ~ | ~ | ~ |
- The paper also provides the same estimate in terms of equivalent emissions: partial dieback would be equivalent to the emissions of 30 billion tonnes of carbon, while total dieback would be equivalent to 75 billion tonnes of carbon
- The paper also provides the same estimate in terms of emissions: between 125 and 250 billion tonnes of carbon and between 175 and 350 billion tonnes of carbon equivalent.
Proposed climate tipping element (and tipping point) | Threshold ( °C) | Timescale (years) | Maximum Impact ( °C) | ||||||
---|---|---|---|---|---|---|---|---|---|
Estimated | Minimum | Maximum | Estimated | Minimum | Maximum | Global | Regional | ||
Boreal Permafrost (abrupt thaw) | 1.5 | 1.0 | 2.3 | 200 | 100 | 300 | 0.04 per °C by 2100;0.11 per °C by 2300[T2 1] | ~ | |
Barents Sea Ice (abrupt loss) | 1.6 | 1.5 | 1.7 | 25 | ? | ? | ~ | + | |
Mountain Glaciers (loss) | 2.0 | 1.5 | 3.0 | 200 | 50 | 1k | 0.08 | + | |
Sahel and W.African Monsoon (greening) | 2.8 | 2 | 3.5 | 50 | 10 | 500 | ~ | + | |
Boreal Forest (southern dieoff) | 4.0 | 1.5 | 5.0 | 100 | 50 | ? | net -0.18[T2 2] | -0.5 to -2 | |
Boreal Forest (northern expansion) | 4.0 | 1.5 | 7.2 | 100 | 40 | ? | net +0.14[T2 3] | 0.5-1.0 |
- The paper clarifies that this represents a 50% increase of gradual permafrost thaw: it also provides the same estimate in terms of emissions per each degree of warming: 10 billion tonnes of carbon and 14 billion tonnes of carbon equivalent by 2100, and 25/35 billion tonnes of carbon/carbon equivalent by 2300
- The loss of these forests would be equivalent to the emissions of 52 billion tons of carbon, but this would be more than offset by the area's albedo effect increasing and reflecting more sunlight.
- Extra forest growth here would absorb around 6 billion tons of carbon, but because this area receives a lot of sunlight, this is very minor when compared to reduced albedo, as this vegetation absorbs more heat than the snow-covered ground it moves into.
Greenland ice sheet disintegration
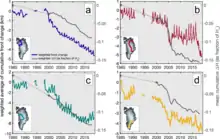
The Greenland ice sheet is the second largest ice sheet in the world, and is three times the size of the American state of Texas.[17] It holds enough water, that if completely melted, could raise sea levels globally by 7.2 metres.[18] Due to global warming, the ice sheet is melting at an accelerating rate, adding almost 1 mm to global sea levels every year.[19] Around half of the ice loss occurs via surface melting, and the remainder occurs at the base of the ice sheet where the ice sheet touches the sea, by calving (breaking off) icebergs from its margins.[20]
The Greenland ice sheet has a tipping point because of the melt-elevation feedback. Surface melting reduces the height of the ice sheet. As air at a lower altitude is warmer, the ice sheet is then exposed to warmer temperatures, accelerating the melt.[21] A 2021 analysis of sub-glacial sediment at the bottom of a 1.4 km Greenland ice core finds that the Greenland ice sheet melted away at least once during the last million years, and therefore strongly suggests that its tipping point is below the 2.5 °C maximum positive temperature excursion over that period.[22][23] There is some evidence that the Greenland ice sheet is losing stability, and getting close to a tipping point.[21]
West Antarctic ice sheet disintegration

The West Antarctic Ice Sheet (WAIS) is a large ice sheet in Antarctica; in places more than 4 kilometres thick. It sits on bedrock mostly below sea level.[24] As such, it is in contact with the heat from the ocean which makes it vulnerable to fast and irreversible ice loss. A tipping point could be reached if thinning or collapse of the WAIS's ice shelves triggers a feedback loop that leads to rapid and irreversible loss of its ice into the ocean. If completely melted, the ice sheet would contribute around 3.3 metres of sea level rise.[10]
Ice loss from the WAIS is accelerating.[25] The paleo record suggests that during the past few hundred thousand years, the WAIS largely disappeared in response to similar levels of warming and CO2 emission scenarios projected for the next few centuries.[26]
Like with the other ice sheets, there is a counteracting negative feedback - greater warming also intensifies the effects of climate change on the water cycle, which result in an increased precipitation over the ice sheet in the form of snow during the winter, which would freeze on the surface, and this increase in the surface mass balance (SMB) counteracts some fraction of the ice loss. In the IPCC Fifth Assessment Report, it was suggested that this effect could potentially overpower increased ice loss under the higher levels of warming and result in small net ice gain, but by the time of the IPCC Sixth Assessment Report, improved modelling had proven that the glacier breakup would consistently accelerate at a faster rate.[27][28]
North Subpolar Gyre
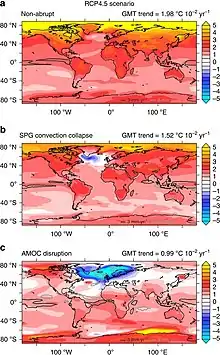
Some climate models indicate that the deep convection in Labrador-Irminger Seas could collapse under certain global warming scenarios, which would then collapse the entire circulation in the North subpolar gyre. It is considered unlikely to recover even if the temperature is returned to a lower level, making it an example of a climate tipping point. This would result in rapid cooling, with implications for economic sectors, agriculture industry, water resources and energy management in Western Europe and the East Coast of the United States.[29] Frajka-Williams et al. 2017 pointed out that recent changes in cooling of the subpolar gyre, warm temperatures in the subtropics and cool anomalies over the tropics, increased the spatial distribution of meridional gradient in sea surface temperatures, which is not captured by the AMO Index.[30]
A 2021 study found that this collapse occurs in only four CMIP6 models out of 35 analyzed. However, only 11 models out of 35 can simulate North Atlantic Current with a high degree of accuracy, and this includes all four models which simulate collapse of the subpolar gyre. As the result, the study estimated the risk of an abrupt cooling event over Europe caused by the collapse of the current at 36.4%, which is lower than the 45.5% chance estimated by the previous generation of models [31] In 2022, a paper suggested that previous disruption of subpolar gyre was connected to the Little Ice Age.[32]East Antarctic ice sheet disintegration
East Antarctic ice sheet is the largest and thickest ice sheet on Earth, with the maximum thickness of 4,800 m. A complete disintegration would raise the global sea levels by 53.3 m, but this may not occur until global warming of 10 degrees Celsius, while the loss of two-thirds of its volume may require at least 6 degrees of warming to trigger.[33] Its melt would also occur over a longer timescale than the loss of any other ice on the planet, taking no less than 10,000 years to finish. However, the subglacial basin portions of the East Antarctic ice sheet may be vulnerable to tipping at lower levels of warming.[5] The Wilkes Basin is of particular concern, as it holds enough ice to raise sea levels by about 3 to 4 metres.[1]

Amazon rainforest dieback
The Amazon rainforest is the largest tropical rainforest in the world. It is twice as big as India and spans nine countries in South America. It produces around half of its own rainfall by recycling moisture through evaporation and transpiration as air moves across the forest.[10] When forest is lost via climate change (droughts and fires) or deforestation, there will be less rain and more trees will die. Eventually, large parts of the rainforest may die off and transform into a dry savanna landscape.[35] In 2022, a study reported that the rainforest has been losing resilience since the early 2000s. Resiliency is measured by recovery-time from short-term perturbations. This delayed return to equilibrium of the rainforest is termed critical slowing down. The observed loss of resilience reinforces the theory that the rainforest is approaching a critical transition.[36][37]
Permafrost thaw
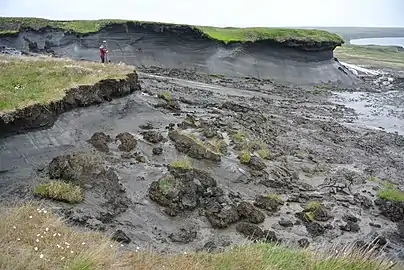
Perennially frozen ground, or permafrost, covers large fractions of land – mainly in Siberia, Alaska, northern Canada and the Tibetan plateau – and can be up to a kilometre thick.[38][10] Subsea permafrost up to 100 metres thick also occurs on the sea floor under part of the Arctic Ocean.[39] This frozen ground holds vast amounts of carbon from plants and animals that died and decomposed over thousands of years. Scientists believe there is nearly twice as much carbon in permafrost than is present in Earth's atmosphere.[39] As the climate warms and the permafrost begins to thaw, carbon dioxide and methane are released into the atmosphere. With higher temperatures, microbes become active and decompose the biological material in the permafrost. This could happen rapidly, or over longer timespans, and the loss would be irreversible. Because CO2 and methane are both greenhouse gases, they act as a self-reinforcing feedback on permafrost melt.[40][41]
Atlantic Meridional Overturning Circulation
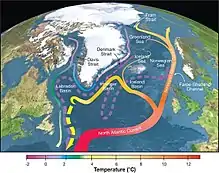
The Atlantic Meridional Overturning Circulation (AMOC), also known as the Gulf Stream System, is a large system of ocean currents.[42][43] It is driven by differences in the density of water; colder and more salty water is heavier than warmer fresh water.[43] The AMOC acts as a conveyor belt, sending warm surface water from the tropics north, and carrying cold fresh water back south.[42] As warm water flows northwards, some evaporates which increases salinity. It also cools when it is exposed to cooler air. Cold, salty water is more dense and slowly begins to sink. Several kilometres below the surface, cold, dense water begins to move south.[43] Increased rainfall and the melting of ice due to global warming dilutes the salty surface water, and warming further decreases its density. The lighter water is less able to sink, slowing down the circulation.[10]
Theory, simplified models, and reconstructions of abrupt changes in the past suggest the AMOC has a tipping point. If freshwater input from melting glaciers reaches a certain threshold, it could collapse into a state of reduced flow. Even after melting stops, the AMOC may not return to its current state. It is unlikely that the AMOC will tip in the 21st century,[44] but it may do so before 2300 if greenhouse gas emissions are very high. A weakening of 24% to 39% is expected depending on greenhouse emissions, even without tipping behaviour.[45] If the AMOC does shut down, a new stable state could emerge that lasts for thousands of years, possibly triggering other tipping points.[10]
In 2021, a study which used a "primitive" finite-difference ocean model estimated that AMOC collapse could be invoked by a sufficiently fast increase in ice melt even if it never reached the common thresholds for tipping obtained from slower change. Thus, it implied that the AMOC collapse is more likely than what is usually estimated by the complex and large-scale climate models.[46] Another 2021 study found early-warning signals in a set of AMOC indices, suggesting that the AMOC may be close to tipping.[47] However, it was contradicted by another study published in the same journal the following year, which found a "largely stable" AMOC which had so far not been affected by climate change beyond its own natural variability.[48] Two more studies published in 2022 have also suggested that the modelling approaches commonly used to evaluate AMOC appear to overestimate the risk of its collapse.[49][50]
Arctic sea ice
Arctic sea ice was once identified as a potential tipping element. The loss of sunlight-reflecting sea ice during summer exposes the (dark) ocean, which would warm. Arctic sea ice cover is likely to melt entirely under even relatively low levels of warming, and it was hypothesized that this could eventually transfer enough heat to the ocean to prevent sea ice recovery even if the global warming is reversed. Modelling now shows that this heat transfer during the Arctic summer does not overcome the cooling and the formation of new ice during the Arctic winter. As such, the loss of Arctic ice during the summer is not a tipping point for as long as the Arctic winter remains cool enough to enable the formation of new Arctic sea ice.[51][52] However, if the higher levels of warming prevent the formation of new Arctic ice even during winter, then this change may become irreversible. Consequently, Arctic Winter Sea Ice is included as a potential tipping point in a 2022 assessment.[5]
Additionally, the same assessment argued that while the rest of the ice in the Arctic Ocean may recover from a total summertime loss during the winter, ice cover in the Barents Sea may not reform during the winter even below 2 degrees of warming.[5] This is because the Barents Sea is already the fastest-warming part of the Arctic: in 2021-2022 it was found that while the warming within the Arctic Circle has already been nearly four times faster than the global average since 1979,[53][54] Barents Sea warmed up to seven times faster than the global average.[55][56] This tipping point matters because of the decade-long history of research into the connections between the state of Barents-Kara Sea ice and the weather patterns elsewhere in Eurasia.[57][58][59][60][61]
Coral reef die-off
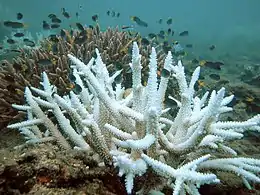
Around 500 million people around the world depend on coral reefs for food, income, tourism and coastal protection.[62] Since the 1980s, this is being threatened by the increase in sea surface temperatures which is triggering mass bleaching of coral, especially in sub-tropical regions.[63] A sustained ocean temperature spike of 1 °C above average is enough to cause bleaching.[64] Under heat stress, corals expel the small colourful algae which live in their tissues, which causes them to turn white. The algae, known as zooxanthellae, have a symbiotic relationship with coral such that without them, the corals slowly die.[65] After these zooxanthellae have disappeared, the corals are vulnerable to a transition towards a seaweed-dominated ecosystem, making it very difficult to shift back to a coral-dominated ecosystem.[66] The IPCC estimates that by the time temperatures have risen to 1.5 °C above pre-industrial times, between 70% and 90% of coral reefs that exist today will disappear; and that if the world warms by 2 °C, they will become extremely rare.[67]
Mountain glaciers

Mountain glaciers are the largest repository of land-bound ice after the Greenland and the Antarctica ice sheets, and they are also undergoing melting as the result of climate change. A glacier tipping point is when it enters a disequilibrium state with the climate and will melt away unless the temperatures go down.[68][69] Examples include glaciers of the North Cascade Range, where even in 2005 67% of the glaciers observed were in disequilibrium and will not survive the continuation of the present climate,[70] or the French Alps, where The Argentière and Mer de Glace glaciers are expected to disappear completely by end of the 21st century if current climate trends persist.[71]
The absolute largest amount of glacier ice is located in the Hindu Kush Himalaya region, which is colloquially known as the Earth's "Third Pole" as the result. It is believed that one third of that ice will be lost by 2100 even if the warming is limited to 1.5 °C, while the "intermediate" and "severe" climate change scenarios (RCP 4.5 and 8.5) are likely to lead to the losses of 50% and >67% of the region's glaciers over the same timeframe. Glacier melt is projected to accelerate regional river flows until the amount of meltwater peaks around 2060, going into an irreversible decline afterwards. Since regional precipitation will continue to increase even as the glacier meltwater contribution declines, annual river flows are only expected to diminish in the western basins where contribution from the monsoon is low: however, irrigation and hydropower generation would still have to adjust to greater interannual variability and lower pre-monsoon flows in all of the region's rivers.[72][73][74]
Sahel greening
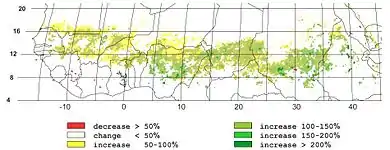
Some simulations of global warming and increased carbon dioxide concentrations have shown a substantial increase in precipitation in the Sahel/Sahara.[75] This and the increased plant growth directly induced by carbon dioxide[76] could lead to an expansion of vegetation into present-day desert, although it would be less extensive than during the mid-Holocene[75] and perhaps accompanied by a northward shift of the desert, i.e. a drying of northernmost Africa.[77] Such a precipitation increase may also reduce the amount of dust originating in Northern Africa,[78] with effects on hurricane activity in the Atlantic and increased threats of hurricane strikes in the Caribbean, the Gulf of Mexico and the East Coast of the United States of America.[79]
The Special Report on Global Warming of 1.5 °C and the IPCC Fifth Assessment Report indicate that global warming will likely result in increased precipitation across most of East Africa, parts of Central Africa and the principal wet season of West Africa, although there is significant uncertainty related to these projections especially for West Africa.[80] In addition, the end of the 20th century drying trend may be due to global warming.[81] On the other hand, West Africa[82] and parts of East Africa may become drier during given seasons and months.[82][81] Currently, the Sahel is becoming greener but precipitation has not fully recovered to levels reached in the mid-20th century.[77]
Climate models have yielded equivocal results about the effects of anthropogenic global warming on the Sahara/Sahel precipitation. Human-caused climate change occurs through different mechanisms than the natural climate change that led to the AHP,[83] in particular through increased inter-hemispheric temperature gradients.[84] The direct effect of heat on plants may be detrimental.[85] Non-linear increases in vegetation cover are also possible.[84] One study in 2003 showed that vegetation intrusions in the Sahara can occur within decades after strong rises in atmospheric carbon dioxide[86] but would not cover more than about 45% of the Sahara.[87] That climate study also indicated that vegetation expansion can only occur if grazing or other perturbations to vegetation growth do not hamper it.[88] On the other hand, increased irrigation and other measures to increase vegetation growth such as the Great Green Wall could enhance it.[85]Boreal forest biome shift
During the last quarter of the twentieth century, the zone of latitude occupied by taiga experienced some of the greatest temperature increases on Earth. Winter temperatures have increased more than summer temperatures. In summer, the daily low temperature has increased more than the daily high temperature.[89] It has been hypothesized that the boreal environments have only a few states which are stable in the long term - a treeless tundra/steppe, a forest with >75% tree cover and an open woodland with ~20% and ~45% tree cover. Thus, continued climate change would be able to force at least some of the presently existing taiga forests into one of the two woodland states or even into a treeless steppe - but it could also shift tundra areas into woodland or forest states as they warm and become more suitable for tree growth.[90]
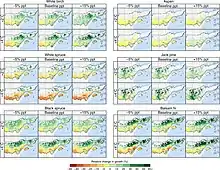
These trends were first detected in the Canadian boreal forests in the early 2010s,[91][92][93][94] and summer warming had also been shown to increase water stress and reduce tree growth in dry areas of the southern boreal forest in central Alaska and portions of far eastern Russia.[95] In Siberia, the taiga is converting from predominantly needle-shedding larch trees to evergreen conifers in response to a warming climate. Subsequent research in Canada found that even in the forests where biomass trends did not change, there was a substantial shift towards the deciduous broad-leaved trees with higher drought tolerance over the past 65 years,[96] and a Landsat analysis of 100,000 undisturbed sites found that the areas with low tree cover became greener in response to warming, but tree mortality (browning) became the dominant response as the proportion of existing tree cover increased.[97] A 2018 study of the seven tree species dominant in the Eastern Canadian forests found that while 2 °C warming alone increases their growth by around 13% on average, water availability is much more important than temperature and further warming of up to 4 °C would result in substantial declines unless matched by increases in precipitation.[98]
A 2021 paper had confirmed that the boreal forests are much more strongly affected by climate change than the other forest types in Canada and projected that most of the eastern Canadian boreal forests would reach a tipping point around 2080 under the RCP 8.5 scenario, which represents the largest potential increase in anthropogenic emissions.[99] Another 2021 study projected that under the "moderate" SSP2-4.5 scenario, boreal forests would experience a 15% worldwide increase in biomass by the end of the century, but this would be more than offset by the 41% biomass decline in the tropics.[100] In 2022, the results of a 5-year warming experiment in North America had shown that the juveniles of tree species which currently dominate the southern margins of the boreal forests fare the worst in response to even 1.5°C or +3.1 °C of warming and the associated reductions in precipitation. While the temperate species which would benefit from such conditions are also present in the southern boreal forests, they are both rare and have slower growth rates.[101]
Equatorial stratocumulus clouds
In 2019, a study employed a large eddy simulation model to estimate that equatorial stratocumulus clouds could break up and scatter when CO2 levels rise above 1,200 ppm (almost three times higher than the current levels, and over 4 times greater than the preindustrial levels). The study estimated that this would cause a surface warming of about 8 degrees Celsius globally and 10 degrees in the subtropics, which would be in addition to at least 4 degrees of warming already caused by such CO2 concentrarions. In addition, stratocumulus clouds would not reform until the CO2 concentrations drop to a much lower level.[102] It was suggested that this finding could help explain past episodes of unusually rapid warming such as Paleocene-Eocene Thermal Maximum[103] However, because large eddy simulation models are simpler and smaller-scale than the general circulation models used for climate projections, with limited representation of atmospheric processes like subsidence, this finding is currently considered speculative. Additionally, CO2 concentrations would only reach 1,200 ppm if the world follows Representative Concentration Pathway 8.5, which represents the highest possible greenhouse gas emission scenario and involves a massive expansion of coal infrastructure. In that case, 1,200 ppm would be passed shortly after 2100.[104]
In 2020, further work from the same authors revealed that in a large eddy simulation, this tipping point cannot be stopped with solar geoengineering: in a hypothetical scenario where very high CO2 emissions continue for a long time but are offset with extensive solar geoengineering, the break-up of stratocumulus clouds is simply delayed until CO2 concentrations hit 1,700 ppm, at which point it would still cause around 5 degrees of unavoidable warming.[105]Formerly considered tipping elements
The possibility that the El Niño–Southern Oscillation (ENSO) is a tipping element had attracted attention in the past.[106] Normally strong winds blow west across the South Pacific Ocean from South America to Australia. Every two to seven years, the winds weaken due to pressure changes and the air and water in the middle of the Pacific warms up, causing changes in wind movement patterns around the globe. This known as El Niño and typically leads to droughts in India, Indonesia and Brazil, and increased flooding in Peru. In 2015/2016, this caused food shortages affecting over 60 million people.[107] El Niño-induced droughts may increase the likelihood of forest fires in the Amazon.[108] The threshold for tipping was estimated to be between 3.5 and 7 °C of global warming in 2016.[109] After tipping, the system would be in a more permanent El Niño state, rather than oscillating between different states. This has happened in Earth's past, in the Pliocene, but the layout of the ocean was significantly different from now.[106] So far, there is no definitive evidence indicating changes in ENSO behaviour,[108] and the IPCC Sixth Assessment Report concluded that it is "virtually certain that the ENSO will remain the dominant mode of interannual variability in a warmer world."[110] Consequently, the 2022 assessment no longer includes it in the list of likely tipping elements.[5]
The Indian summer monsoon is another part of the climate system which was considered suspectible to irreversible collapse in the earlier research.[111] However, more recent research has demonstrated that warming tends to strengthen the Indian monsoon,[112] and it is projected to strengthen in the future.[113]
Methane hydrate deposits in the Arctic were once thought to be vulnerable to a rapid dissociation which would have a large impact on global temperatures, in a dramatic scenario known as a clathrate gun hypothesis. Later research found that it takes millennia for methane hydrates to respond to warming,[114] while methane emissions from the seafloor rarely transfer from the water column into the atmosphere.[115][116][117] IPCC Sixth Assessment Report states "It is very unlikely that gas clathrates (mostly methane) in deeper terrestrial permafrost and subsea clathrates will lead to a detectable departure from the emissions trajectory during this century".[118]
Mathematical theory
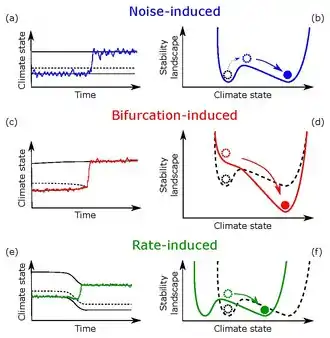
Tipping point behaviour in the climate can be described in mathematical terms. Three types of tipping points have been identified—bifurcation, noise-induced and rate-dependent.[119][120]
Bifurcation-induced tipping
Bifurcation-induced tipping happens when a particular parameter in the climate (for instance a change in environmental conditions or forcing), passes a critical level – at which point a bifurcation takes place – and what was a stable state loses its stability or simply disappears.[120][121] The Atlantic Meridional Overturning Circulation (AMOC) is an example of a tipping element that can show bifurcation-induced tipping. Slow changes to the bifurcation parameters in this system – the salinity and temperature of the water – may push the circulation towards collapse.[122][123]
Many types of bifurcations show hysteresis,[124] which is the dependence of the state of a system on its history. For instance, depending on how warm it was in the past, there can be differing amounts of ice on the poles at the same concentration of greenhouse gases or temperature.[125]
Early warning signals
For tipping points that occur because of a bifurcation, it may be possible to detect whether a system is getting closer to a tipping point, as it becomes less resilient to perturbations on approach of the tipping threshold. These systems display critical slowing down, with an increased memory (rising autocorrelation) and variance. Depending on the nature of the tipping system, there may be other types of early warning signals.[126][127] Abrupt change is not an early warning signal (EWS) for tipping points, as abrupt change can also occur if the changes are reversible to the control parameter.[128][129]
These EWSs are often developed and tested using time series from the paleo record, like sediments, ice caps, and tree rings, where past examples of tipping can be observed.[126][130] It is not always possible to say whether increased variance and autocorrelation is a precursor to tipping, or caused by internal variability, for instance in the case of the collapse of the AMOC.[130] Quality limitations of paleodata further complicate the development of EWSs.[130] They have been developed for detecting tipping due to drought in forests in California,[131] and melting of the Pine Island Glacier in West Antarctica,[129] among other systems. Using early warning signals (increased autocorrelation and variance of the melt rate time series), it has been suggested that the Greenland ice sheet is currently losing resilience, consistent with modelled early warning signals of the ice sheet.[132]
Human-induced changes in the climate system may be too fast for early warning signals to become evident, especially in systems with inertia.[133]
Noise-induced tipping
Noise-induced tipping is the transition from one state to another due to random fluctuations or internal variability of the system. Noise-induced transitions do not show any of the early warning signals which occur with bifurcations. This means they are unpredictable because the underlying potential does not change. Because they are unpredictable, such occurrences are often described as a "one-in-x-year" event.[134] An example is the Dansgaard–Oeschger events during the last ice age, with 25 occurrences of sudden climate fluctuations over a 500 year period.[135]
Rate-induced tipping
Rate-induced tipping occurs when a change in the environment is faster than the force that restores the system to its stable state.[120] In peatlands, for instance, after years of relative stability, rate-induced tipping can lead to an "explosive release of soil carbon from peatlands into the atmosphere" – sometimes known as "compost bomb instability".[136][137] The AMOC may also show rate-induced tipping: if the rate of ice melt increases too fast, it may collapse, even before the ice melt reaches the critical value where the system would undergo a bifurcation.[138]
Cascading tipping points
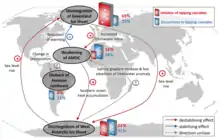
Crossing a threshold in one part of the climate system may trigger another tipping element to tip into a new state. Such sequences of thresholds are called cascading tipping points, an example of a domino effect.[139] Ice loss in West Antarctica and Greenland will significantly alter ocean circulation. Sustained warming of the northern high latitudes as a result of this process could activate tipping elements in that region, such as permafrost degradation, and boreal forest dieback.[1] Thawing permafrost is a threat multiplier because it holds roughly twice as much carbon as the amount currently circulating in the atmosphere.[140] Loss of ice in Greenland likely destabilises the West Antarctic ice sheet via sea level rise, and vice-versa, especially if Greenland were to melt first as West Antarctica is particularly vulnerable to contact with warm sea water.[141]
A 2021 study with three million computer simulations of a climate model showed that nearly one-third of those simulations resulted in domino effects, even when temperature increases were limited to 2 °C – the upper limit set by the Paris Agreement in 2015.[141][142] The authors of the study said that the science of tipping points is so complex that there is great uncertainty as to how they might unfold, but nevertheless, argued that the possibility of cascading tipping points represents "an existential threat to civilisation".[143]
Impacts
Tipping points can have very severe impacts.[1] They can exacerbate current dangerous impacts of climate change, or give rise to new impacts. Some potential tipping points would take place abruptly, such as disruptions to the Indian monsoon, with severe impacts on food security for hundred of millions. Other impacts would likely take place over longer timescales, such as the melt of the ice caps. The 10 m of sea level rise from the combined melt of Greenland and West Antarctica would require moving many cities inland. A collapse of the Atlantic Overturning Circulation would alter Europe radically, and lead to a metre of sea level rise in the North Atlantic.[3] These impacts could happen simultaneously in the case of cascading tipping points.[114] A review of abrupt changes over the last 30,000 years showed that tipping points can lead to a large set of cascading impacts in climate, ecological and social systems. For instance, the abrupt termination of the African humid period cascaded, and desertification and regime shifts led to the retreat of pastoral societies in North Africa and a change of dynasty in Egypt.[130]
A 2021 meta analysis on the potential economic impact of tipping points found that they raise global risk; the medium estimate was that they increase the social cost of carbon by about 25%, with a 10% chance of tipping points more than doubling it. The social cost of carbon reflects the economic damage from carbon emissions.[144] It included a detailed table of the estimated additional impact from each tipping point per every tonne of carbon under the "intermediate" Representative Concentration Pathway 4.5 Since it was written before the publication of the IPCC Sixth Assessment Report and the 2022 assessment of tipping points, it includes some tipping points they no longer consider plausible, like methane hydrates and the Indian summer monsoon, and excludes the more recently identified ones like the Labrador-Irminger circulation.
Tipping point (TP) | Expected SCC (US$/tCO2) | Increase due to TP, % |
---|---|---|
None | 52.03 | — |
Permafrost carbon | 56.41 | 8.4 |
Ocean methane hydrates | 58.85 | 13.1 |
Arctic sea ice/Surface Albedo Feedback | 51.14 | -1.7 |
Amazon | 52.07 | 0.1 |
Greenland Ice Sheet | 52.97 | 1.8 |
West Antarctic Ice Sheet | 53.57 | 2.9 |
AMOC | 51.28 | -1.4 |
Indian summer monsoon | 52.70 | 1.3 |
All TPs | 64.80 | 24.5 |
Σ main effects, all TPs | — | 24.5 |
All costly TPs | 67.05 | 28.9 |
Σ main effects, costly TPs only | — | 27.6 |
In 2022, this assessment was severely criticized by a group of scientists including Steve Keen and Timothy Lenton, who considered those values, as well as the paper's suggestion that tipping points activated at 6 °C of global warming would reduce global per capita consumption by around 1.4%, to be an enormous underestimate.[145] The authors of the assessment responded to this criticism by noting that their assessment should be treated as the starting point in economic assessment of tipping points rather than the final word, and since most of the literature included in their meta-analysis lacks the ability to estimate nonmarket climate damages, their numbers are likely to be underestimates. They have also noted that if climate change is assumed to impact the economic growth rate rather than the base level of economic activity (which they consider unlikely, but still a possibility) then the social cost of carbon becomes nearly 100 times larger, while the impact of tipping points becomes around 3 times larger.[146]
Runaway greenhouse effect
A runaway greenhouse effect is a tipping point so extreme that oceans evaporate[147] and the water vapour escapes to space, an irreversible climate state that happened on Venus.[148] A runaway greenhouse effect has virtually no chance of being caused by people.[149]
Venus-like conditions on the Earth require a large long-term forcing that is unlikely to occur until the sun brightens by a few tens of percents, which will take a few billion years.[150]
See also
- Greenhouse and icehouse Earth
- Climate sensitivity
- Planetary boundaries
- World Scientists' Warning to Humanity
References
- Lenton, Tim; Rockström, Johan; Gaffney, Owen; Rahmstorf, Stefan; Richardson, Katherine; Steffen, Will; Schellnhuber, Hans Joachim (2019). "Climate tipping points – too risky to bet against". Nature. 575 (7784): 592–595. Bibcode:2019Natur.575..592L. doi:10.1038/d41586-019-03595-0. PMID 31776487.
- "Climate change driving entire planet to dangerous "global tipping point"". National Geographic. 27 November 2019. Retrieved 17 July 2022.
- Lenton, Tim (2021). "Tipping points in the climate system". Weather. 76 (10): 325–326. Bibcode:2021Wthr...76..325L. doi:10.1002/wea.4058. ISSN 0043-1656. S2CID 238651749.
- Armstrong McKay, David; Abrams, Jesse; Winkelmann, Ricarda; Sakschewski, Boris; Loriani, Sina; Fetzer, Ingo; Cornell, Sarah; Rockström, Johan; Staal, Arie; Lenton, Timothy (9 September 2022). "Exceeding 1.5°C global warming could trigger multiple climate tipping points". Science. 377 (6611). doi:10.1126/science.abn7950. ISSN 0036-8075.
- Armstrong McKay, David (9 September 2022). "Exceeding 1.5°C global warming could trigger multiple climate tipping points – paper explainer". climatetippingpoints.info. Retrieved 2 October 2022.
- Brovkin, Victor; Brook, Edward; Williams, John W.; Bathiany, Sebastian; Lenton, Tim; Barton, Michael; DeConto, Robert M.; Donges, Jonathan F.; Ganopolski, Andrey; McManus, Jerry; Praetorius, Summer (2021). "Past abrupt changes, tipping points and cascading impacts in the Earth system". Nature Geoscience. 14 (8): 550–558. doi:10.1038/s41561-021-00790-5. ISSN 1752-0908. S2CID 236504982.
- Ripple, William J; Wolf, Christopher; Newsome, Thomas M.; Gregg, Jillian W.; Lenton, Tim; Palomo, Ignacio; Eikelboom, Jasper A. J.; Law, Beverly E.; Huq, Saleemul; Duffy, Philip B.; Rockström, Johan (28 July 2021). "World Scientists' Warning of a Climate Emergency 2021". BioScience. 71 (biab079): 894–898. doi:10.1093/biosci/biab079. hdl:1808/30278. ISSN 0006-3568.
- Wunderling, Nico; Donges, Jonathan F.; Kurths, Jürgen; Winkelmann, Ricarda (3 June 2021). "Interacting tipping elements increase risk of climate domino effects under global warming". Earth System Dynamics. 12 (2): 601–619. Bibcode:2021ESD....12..601W. doi:10.5194/esd-12-601-2021. ISSN 2190-4979. S2CID 236247596. Archived from the original on 4 June 2021. Retrieved 4 June 2021.
- "IPCC AR6 WG1 Ch4" (PDF). p. 95. Archived (PDF) from the original on 5 September 2021. Retrieved 14 November 2021.
- "Explainer: Nine "tipping points" that could be triggered by climate change". Carbon Brief. 10 February 2020. Retrieved 16 July 2022.
- Heinze, Christoph; Blenckner, Thorsten; Martins, Helena; Rusiecka, Dagmara; Döscher, Ralf; Gehlen, Marion; Gruber, Nicolas; Holland, Elisabeth; Hov, Øystein; Joos, Fortunat; Matthews, John Brian Robin (2021). "The quiet crossing of ocean tipping points". Proceedings of the National Academy of Sciences. 118 (9): e2008478118. doi:10.1073/pnas.2008478118. ISSN 0027-8424. PMC 7936299. PMID 33619085.
- "Glossary — Special Report on the Ocean and Cryosphere in a Changing Climate". Archived from the original on 16 August 2021. Retrieved 10 July 2021.
- Defined in IPCC_AR6_WGI_Chapter_04 Archived 5 September 2021 at the Wayback Machine, p.95, line 34.
- "Critical measures of global heating reaching tipping point, study finds". the Guardian. 28 July 2021.
- Ripple, William J; Wolf, Christopher; Newsome, Thomas M.; Gregg, Jillian W.; Lenton, Tim; Palomo, Ignacio; Eikelboom, Jasper A. J.; Law, Beverly E.; Huq, Saleemul; Duffy, Philip B.; Rockström, Johan (28 July 2021). "World Scientists' Warning of a Climate Emergency 2021". BioScience. 71 (biab079): 894–898. doi:10.1093/biosci/biab079. hdl:1808/30278. ISSN 0006-3568.
- Baker, Harry (15 September 2022). "Climate "points of no return" may be much closer than we thought". livescience.com. Retrieved 18 September 2022.
- "Quick Facts on Ice Sheets". National Snow and Ice Data Center. Retrieved 17 July 2022.
- "New climate models suggest faster melting of the Greenland Ice Sheet". World Economic Forum. Retrieved 17 July 2022.
- Scambos, Ted; Straneo, Fiamma; Tedesco, Marco (2021). "How fast is the Greenland ice sheet melting?". Arctic, Antarctic, and Alpine Research. 53 (1): 221–222. doi:10.1080/15230430.2021.1946241. ISSN 1523-0430. S2CID 242536272.
- Todd, Joe; Christoffersen, Poul; Zwinger, Thomas; Råback, Peter; Chauché, Nolwenn; Benn, Doug; Luckman, Adrian; Ryan, Johnny; Toberg, Nick; Slater, Donald; Hubbard, Alun (2018). "A Full-Stokes 3-D Calving Model Applied to a Large Greenlandic Glacier". Journal of Geophysical Research: Earth Surface. 123 (3): 410–432. doi:10.1002/2017JF004349. S2CID 54546830.
- Boers, Niklas; Rypdal, Martin (2021). "Critical slowing down suggests that the western Greenland Ice Sheet is close to a tipping point". Proceedings of the National Academy of Sciences. 118 (21): e2024192118. doi:10.1073/pnas.2024192118. ISSN 0027-8424. PMC 8166178. PMID 34001613.
- Garric, Audrey (15 March 2021). "La calotte glaciaire du Groenland a déjà fondu au moins une fois au cours du dernier million d'années". Le Monde.
- Christ, Andrew J.; Bierman, Paul R.; Schaefer, Joerg M.; Dahl-Jensen, Dorthe; Steffensen, Jørgen P.; Corbett, Lee B.; Peteet, Dorothy M.; Thomas, Elizabeth K.; Steig, Eric J.; Rittenour, Tammy M.; Tison, Jean-Louis; Blard, Pierre-Henri; Perdrial, Nicolas; Dethier, David P.; Lini, Andrea; Hidy, Alan J.; Caffee, Marc W.; Southon, John (30 March 2021). "A multimillion-year-old record of Greenland vegetation and glacial history preserved in sediment beneath 1.4 km of ice at Camp Century". Proceedings of the National Academy of Sciences of the United States. 118 (13): e2021442118. Bibcode:2021PNAS..11821442C. doi:10.1073/pnas.2021442118. PMC 8020747. PMID 33723012.
- Fretwell, P.; Pritchard, H. D.; Vaughan, D. G.; Bamber, J. L.; Barrand, N. E.; Bell, R.; Bianchi, C.; Bingham, R. G.; Blankenship, D. D.; Casassa, G.; Catania, G. (28 February 2013). "Bedmap2: improved ice bed, surface and thickness datasets for Antarctica". The Cryosphere. 7 (1): 375–393. doi:10.5194/tc-7-375-2013. ISSN 1994-0416. S2CID 13129041.
- Shepherd, Andrew; Ivins, Erik; Rignot, Eric; Smith, Ben; van den Broeke, Michiel; Velicogna, Isabella; Whitehouse, Pippa; Briggs, Kate; Joughin, Ian; Krinner, Gerhard; Nowicki, Sophie (2018). "Mass balance of the Antarctic Ice Sheet from 1992 to 2017". Nature. 558 (7709): 219–222. doi:10.1038/s41586-018-0179-y. hdl:2268/225208. ISSN 1476-4687. PMID 29899482. S2CID 186244208.
- Joughin, Ian; Alley, Richard B. (2011). "Stability of the West Antarctic ice sheet in a warming world". Nature Geoscience. 4 (8): 506–513. doi:10.1038/ngeo1194. ISSN 1752-0908.
- Justin Gillis (March 22, 2016) "Scientists Warn of Perilous Climate Shift Within Decades, Not Centuries" New York Times
- Fox-Kemper, B.; Hewitt, H.T.; Xiao, C.; Aðalgeirsdóttir, G.; Drijfhout, S.S.; Edwards, T.L.; Golledge, N.R.; Hemer, M.; Kopp, R.E.; Krinner, G.; Mix, A. (2021). Masson-Delmotte, V.; Zhai, P.; Pirani, A.; Connors, S.L.; Péan, C.; Berger, S.; Caud, N.; Chen, Y.; Goldfarb, L. (eds.). "Chapter 9: Ocean, Cryosphere and Sea Level Change" (PDF). Climate Change 2021: The Physical Science Basis. Contribution of Working Group I to the Sixth Assessment Report of the Intergovernmental Panel on Climate Change. Cambridge University Press, Cambridge, UK and New York, NY, USA: 1270–1272.
- Sgubin; et al. (2017). "Abrupt cooling over the North Atlantic in modern climate models". Nature Communications. 8. Bibcode:2017NatCo...8.....S. doi:10.1038/ncomms14375. PMC 5330854. PMID 28198383.
- Eleanor Frajka-Williams; Claudie Beaulieu; Aurelie Duchez (2017). "Emerging negative Atlantic Multidecadal Oscillation index in spite of warm subtropics". Scientific Reports. 7 (1): 11224. Bibcode:2017NatSR...711224F. doi:10.1038/s41598-017-11046-x. PMC 5593924. PMID 28894211.
- Swingedouw, Didier; Bily, Adrien; Esquerdo, Claire; Borchert, Leonard F.; Sgubin, Giovanni; Mignot, Juliette; Menary, Matthew (2021). "On the risk of abrupt changes in the North Atlantic subpolar gyre in CMIP6 models". Annals of the New York Academy of Sciences. 1504 (1): 187–201. Bibcode:2021NYASA1504..187S. doi:10.1111/nyas.14659. PMID 34212391. S2CID 235712017.
- Arellano-Nava, Beatriz; Halloran, Paul R.; Boulton, Chris A.; Scourse, James; Butler, Paul G.; Reynolds, David J.; Lenton, Timothy (25 August 2022). "Destabilisation of the Subpolar North Atlantic prior to the Little Ice Age". Nature Communications. 13 (1): 5008. Bibcode:2022NatCo..13.5008A. doi:10.1038/s41467-022-32653-x. PMC 9411610. PMID 36008418.
- Garbe, Julius; Albrecht, Torsten; Levermann, Anders; Donges, Jonathan F.; Winkelmann, Ricarda (2020). "The hysteresis of the Antarctic Ice Sheet". Nature (585): 538–544. doi:10.1038/s41586-020-2727-5.
- "Amazon Against the Clock: A Regional Assessment on Where and How to Protect 80% by 2025" (PDF). Amazon Watch. September 2022. p. 8. Archived (PDF) from the original on 10 September 2022.
Graphic 2: Current State of the Amazon by country, by percentage / Source: RAISG (Red Amazónica de Información Socioambiental Georreferenciada) Elaborated by authors.
- Amigo, Ignacio (2020). "When will the Amazon hit a tipping point?". Nature. 578 (7796): 505–507. doi:10.1038/d41586-020-00508-4. PMID 32099130. S2CID 211265824.
- "Climate crisis: Amazon rainforest tipping point is looming, data shows". The Guardian. 7 March 2022. Retrieved 18 April 2022.
- Boulton, Chris A.; Lenton, Tim; Boers, Niklas (March 2022). "Pronounced loss of Amazon rainforest resilience since the early 2000s". Nature Climate Change. 12 (3): 271–278. Bibcode:2022NatCC..12..271B. doi:10.1038/s41558-022-01287-8. ISSN 1758-6798. S2CID 247255222.
- Zhang, T.; Barry, R. G.; Knowles, K.; Heginbottom, J. A.; Brown, J. (2008). "Statistics and characteristics of permafrost and ground-ice distribution in the Northern Hemisphere". Polar Geography. 31 (1–2): 47–68. doi:10.1080/10889370802175895. ISSN 1088-937X. S2CID 129146972.
- "Where is Frozen Ground?". National Snow and Ice Data Center. Retrieved 17 July 2022.
- Viglione, Giuliana (14 March 2022). "'Imminent' tipping point threatening Europe's permafrost peatlands". Carbon Brief. Retrieved 16 July 2022.
- Fewster, Richard E.; Morris, Paul J.; Ivanovic, Ruza F.; Swindles, Graeme T.; Peregon, Anna M.; Smith, Christopher J. (2022). "Imminent loss of climate space for permafrost peatlands in Europe and Western Siberia". Nature Climate Change. 12 (4): 373–379. doi:10.1038/s41558-022-01296-7. ISSN 1758-6798. S2CID 247440316. Archived from the original on 21 February 2022.
- Potsdam Institute for Climate Impact Research. "Gulf Stream System at its weakest in over a millennium". ScienceDaily. Retrieved 17 July 2022.
- "What is the Atlantic Meridional Overturning Circulation?". Met Office. Retrieved 26 November 2021.
- "Risk management of climate thresholds and feedbacks: Atlantic Meridional Overturning Circulation (AMOC)" (PDF). Met Office. December 2019. Retrieved 25 November 2020.
- Fox-Kemper, Baylor; Hewitt, Helene T.; Xiao, Cunde; Aðalgeirsdóttir, Guðfinna; et al. (2021). "Chapter 9: Ocean, cryosphere, and sea level change" (PDF). IPCC AR6 WG1. Section 9.2.3.1.
- Lohmann, Johannes; Ditlevsen, Peter D. (2 March 2021). "Risk of tipping the overturning circulation due to increasing rates of ice melt". Proceedings of the National Academy of Sciences. 118 (9): e2017989118. doi:10.1073/pnas.2017989118. ISSN 0027-8424. PMC 7936283. PMID 33619095.
- Boers, Niklas (2021). "Observation-based early-warning signals for a collapse of the Atlantic Meridional Overturning Circulation". Nature Climate Change. 11 (8): 680–688. doi:10.1038/s41558-021-01097-4. ISSN 1758-6798. S2CID 236930519.
- Latif, Mojib; Sun, Jing; Visbeck, Martin; Bordbar, M. Hadi (25 April 2022). "Natural variability has dominated Atlantic Meridional Overturning Circulation since 1900". Nature Climate Change. 12: 455–460. doi:10.1038/s41558-022-01342-4.
- He, Feng; Clark, Peter U. (7 April 2022). "Freshwater forcing of the Atlantic Meridional Overturning Circulation revisited". Nature Climate Change. 12: 449–454. doi:10.1038/s41558-022-01328-2.
- Kim, Soong-Ki; Kim, Hyo-Jeong; Dijkstra, Henk A.; An, Soon-Il (11 February 2022). "Slow and soft passage through tipping point of the Atlantic Meridional Overturning Circulation in a changing climate". npj Climate and Atmospheric Science. 5 (13). doi:10.1038/s41612-022-00236-8.
- "Does Arctic sea ice have a tipping point?". National Snow and Ice Data Center. 17 December 2021. Retrieved 19 July 2022.
- Arias, Paola A.; Bellouin, Nicolas; Coppola, Erika; Jones, Richard G.; et al. (2021). "Technical Summary" (PDF). IPCC AR6 WG1. p. 76.
- Rantanen, Mika; Karpechko, Alexey Yu; Lipponen, Antti; Nordling, Kalle; Hyvärinen, Otto; Ruosteenoja, Kimmo; Vihma, Timo; Laaksonen, Ari (11 August 2022). "The Arctic has warmed nearly four times faster than the globe since 1979". Communications Earth & Environment. 3 (1): 1–10. doi:10.1038/s43247-022-00498-3. ISSN 2662-4435. S2CID 251498876.
- "The Arctic is warming four times faster than the rest of the world". Science Magazine. 14 December 2021. Retrieved 6 October 2022.
- Isaksen, Ketil; Nordli, Øyvind; et al. (15 June 2022). "Exceptional warming over the Barents area". Scientific Reports. 12. doi:10.1038/s41598-022-13568-5.
- Damian Carrington (15 June 2022). "New data reveals extraordinary global heating in the Arctic". The Guardian. Retrieved 7 October 2022.
- Petoukhov, Vladimir; Semenov, Vladimir A. (2010). "A link between reduced Barents-Kara sea ice and cold winter extremes over northern continents" (PDF). Journal of Geophysical Research. 115 (D21): D21111. Bibcode:2010JGRD..11521111P. doi:10.1029/2009JD013568.
- He, Shengping; Gao, Yongqi; Furevik, Tore; Wang, Huijun; Li, Fei (16 December 2017). "Teleconnection between sea ice in the Barents Sea in June and the Silk Road, Pacific–Japan and East Asian rainfall patterns in August". Advances in Atmospheric Sciences. 35: 52–64. doi:10.1007/s00376-017-7029-y.
- Zhang, Ruonan; Screen, James A. (16 June 2021). "Diverse Eurasian Winter Temperature Responses to Barents-Kara Sea Ice Anomalies of Different Magnitudes and Seasonality". Geophysical Research Letters. 48 (13). doi:10.1029/2021GL092726.
- Song, Mirong; Wang, Zhao-Yin; Zhu, Zhu; Liu, Ji-Ping (August 2021). "Nonlinear changes in cold spell and heat wave arising from Arctic sea-ice loss". Advances in Climate Change Research. 12 (4): 553–562. doi:10.1016/j.accre.2021.08.003.
- Sun, Jianqi; Liu, Sichang; Cohen, Judah; Yu, Shui (2 August 2022). "Influence and prediction value of Arctic sea ice for spring Eurasian extreme heat events". Communications Earth & Environment. 3. doi:10.1038/s43247-022-00503-9.
- Gibbens, Sarah (4 June 2020). "The world's coral reefs are dying—here's how scientists plan to save them". National Geographic. Retrieved 17 July 2022.
- Hughes, Terry P.; Kerry, James T.; Álvarez-Noriega, Mariana; Álvarez-Romero, Jorge G.; Anderson, Kristen D.; Baird, Andrew H.; Babcock, Russell C.; Beger, Maria; Bellwood, David R.; Berkelmans, Ray; Bridge, Tom C. (2017). "Global warming and recurrent mass bleaching of corals". Nature. 543 (7645): 373–377. doi:10.1038/nature21707. hdl:20.500.11937/52828. ISSN 1476-4687. PMID 28300113. S2CID 205254779.
- Worland, Justin. "Explore This Coral Reef Before it Disappears". Time. Retrieved 17 July 2022.
- Gilmour, James Paton; Green, Rebecca (21 May 2019). "'Bright white skeletons': some Western Australian reefs have the lowest coral cover on record". The Conversation. Retrieved 17 July 2022.
- Holbrook, Sally J.; Schmitt, Russell J.; Adam, Thomas C.; Brooks, Andrew J. (2016). "Coral Reef Resilience, Tipping Points and the Strength of Herbivory". Scientific Reports. 6 (1): 35817. doi:10.1038/srep35817. ISSN 2045-2322. PMC 5090207. PMID 27804977.
- IPCC (2018). "Summary for Policymakers" (PDF). Global warming of 1.5°C: An IPCC Special Report on the impacts of global warming of 1.5°C. p. 8.
- Hubbard, Bryn; Neil F. Glasser (20 May 2005). Field Techniques in Glaciology and Glacial Geomorphology. Wiley. pp. 179–198. ISBN 978-0470844274. Retrieved 23 November 2020.
- Pelto, M.S. (2010). "Forecasting temperate alpine glacier survival from accumulation zone observations". The Cryosphere. 4 (1): 67–75. Bibcode:2010TCry....4...67P. doi:10.5194/tc-4-67-2010. Retrieved 23 November 2020.
- Mauri S. Pelto. "North Cascade Glacier Terminus Behavior". Nichols College. Retrieved 7 August 2016.
- Vaughn, Adam (18 September 2019). "Special report: How climate change is melting France's largest glacier". New Scientist. Retrieved 3 February 2021.
- Damian Carrington (4 February 2019). "A third of Himalayan ice cap doomed, finds report". Retrieved 20 October 2022.
- Bolch, Tobias; Shea, Joseph M.; Liu, Shiyin; Azam, Farooq M.; Gao, Yang; Gruber, Stephan; Immerzeel, Walter W.; Kulkarni, Anil; Li, Huilin; Tahir, Adnan A.; Zhang, Guoqing; Zhang, Yinsheng (5 January 2019). "Status and Change of the Cryosphere in the Extended Hindu Kush Himalaya Region". The Hindu Kush Himalaya Assessment: Mountains, Climate Change, Sustainability and People. pp. 209–255.
- Scott, Christopher A.; Zhang, Fan; Mukherji, Aditi; Immerzeel, Walter; Mustafa, Daanish; Bharati, Luna (5 January 2019). "Water in the Hindu Kush Himalaya". The Hindu Kush Himalaya Assessment: Mountains, Climate Change, Sustainability and People. pp. 257–299. doi:10.1007/978-3-319-92288-1_8.
- Renssen et al. 2003, p. 4.
- Pausata et al. 2020, p. 236.
- Brooks et al. 2007, p. 267.
- Donnelly et al. 2017, p. 6221.
- Donnelly et al. 2017, p. 6225.
- IPCC 2014, pp. 16–17.
- IPCC 2014, p. 11.
- "Impacts of 1.5°C of Global Warming on Natural and Human Systems". IPCC. 23 May 2019. p. 197. Retrieved 29 December 2018.
- Petoukhov et al. 2003, p. 100.
- Pausata et al. 2020, p. 242.
- Pausata et al. 2020, p. 244.
- Petoukhov et al. 2003, p. 114.
- Petoukhov et al. 2003, p. 99.
- Petoukhov et al. 2003, p. 113.
- Wilmking, M. (9 October 2009). "Coincidence and Contradiction in the Warming Boreal Forest". Geophysical Research Letters. 32 (15): L15715. Bibcode:2005GeoRL..3215715W. doi:10.1029/2005GL023331. Retrieved 14 January 2012.
- Scheffer, Marten; Hirota, Marina; Holmgren, Milena; Van Nes, Egbert H.; Chapin, F. Stuart (26 December 2012). "Thresholds for boreal biome transitions". Proceedings of the National Academy of Sciences. 109 (52): 21384–21389. doi:10.1073/pnas.1219844110. ISSN 0027-8424. PMC 3535627. PMID 23236159.
- Peng, Changhui; Ma, Zhihai; Lei, Xiangdong; Zhu, Qiuan; Chen, Huai; Wang, Weifeng; Liu, Shirong; Li, Weizhong; Fang, Xiuqin; Zhou, Xiaolu (20 November 2011). "A drought-induced pervasive increase in tree mortality across Canada's boreal forests". Nature Climate Change. 1: 467–471. doi:10.1038/nclimate1293.
- Ma, Zhihai; Peng, Changhui; Zhu, Qiuan; Chen, Huai; Yu, Guirui; Li, Weizhong; Zhou, Xiaolu; Wang, Weifeng; Zhang, Wenhua (30 January 2012). "Regional drought-induced reduction in the biomass carbon sink of Canada's boreal forests". Biological Sciences. 109 (7): 2423–2427. doi:10.1073/pnas.1111576109.
- Chen, Han Y. H.; Luo, Yong (2 July 2015). "Net aboveground biomass declines of four major forest types with forest ageing and climate change in western Canada's boreal forests". Global Change Biology. 21 (10): 3675–3684. doi:10.1111/gcb.12994.
- Sulla-Menashe, Damien; Woodcock, Curtis E; Friedl, Mark A (4 January 2018). "Canadian boreal forest greening and browning trends: an analysis of biogeographic patterns and the relative roles of disturbance versus climate drivers". Environmental Research Letters. 13 (1): 014007. doi:10.1088/1748-9326/aa9b88.
- "Boreal Forests and Climate Change - Changes in Climate Parameters and Some Responses, Effects of Warming on Tree Growth on Productive Sites". Archived from the original on 27 July 2011. Retrieved 25 March 2011.
- Hisano, Masumi; Ryo, Masahiro; Chen, Xinli; Chen, Han Y. H. (16 May 2021). "Rapid functional shifts across high latitude forests over the last 65 years". Global Change Biology. 27 (16): 3846–3858. doi:10.1111/gcb.15710.
- Berner, Logan T.; Goetz, Scott J. (24 February 2022). "Satellite observations document trends consistent with a boreal forest biome shift". Global Change Biology. 28 (10): 3846–3858. doi:10.1111/gcb.16121.
- D'Orangeville, Loïc; Houle, Daniel; Duchesne, Louis; Phillips, Richard P.; Bergeron, Yves; Kneeshaw, Daniel (10 August 2018). "Beneficial effects of climate warming on boreal tree growth may be transitory". Nature Communications. 9. doi:10.1038/s41467-018-05705-4.
- Boulanger, Yan; Puigdevall, Jesus Pascual (3 April 2021). "Boreal forests will be more severely affected by projected anthropogenic climate forcing than mixedwood and northern hardwood forests in eastern Canada". Landscape Ecology. 36: 1725–1740. doi:10.1007/s10980-021-01241-7.
- Larjavaara, Markku; Lu, Xiancheng; Chen, Xia; Vastaranta, Mikko (12 October 2021). "Impact of rising temperatures on the biomass of humid old-growth forests of the world". Carbon Balance and Management. 16. doi:10.1186/s13021-021-00194-3.
- Reich, Peter B.; Bermudez, Raimundo; Montgomery, Rebecca A.; Rich, Roy L.; Rice, Karen E.; Hobbie, Sarah E.; Stefanski, Artur (10 August 2022). "Even modest climate change may lead to major transitions in boreal forests". Nature. 608: 540–545. doi:10.1038/s41586-022-05076-3.
- Schneider, Tapio; Kaul, Colleen M.; Pressel, Kyle G. (2019). "Possible climate transitions from breakup of stratocumulus decks under greenhouse warming". Nature Geoscience (12): 163–167. doi:10.1038/s41561-019-0310-1.
- Wolchover, Natalie (25 February 2019). "A World Without Clouds". Quanta Magazine. Retrieved 2 October 2022.
- "Extreme CO2 levels could trigger clouds 'tipping point' and 8C of global warming". Carbon Brief. 25 February 2019. Retrieved 2 October 2022.
- Schneider, Tapio; Kaul, Colleen M.; Pressel, Kyle G. (2020). "Solar geoengineering may not prevent strong warming from direct effects of CO2 on stratocumulus cloud cover". PNAS. 117 (48): 30179–30185. doi:10.1073/pnas.2003730117.
- Wunderling, Nico; Donges, Jonathan F.; Kurths, Jürgen; Winkelmann, Ricarda (3 June 2021). "Interacting tipping elements increase risk of climate domino effects under global warming". Earth System Dynamics. 12 (2): 601–619. Bibcode:2021ESD....12..601W. doi:10.5194/esd-12-601-2021. ISSN 2190-4979. S2CID 236247596.
- "Tipping Points: Why we might not be able to reverse climate change". ClimateScience. Retrieved 17 July 2022.
- Duque-Villegas, Mateo; Salazar, Juan Fernando; Rendón, Angela Maria (2019). "Tipping the ENSO into a permanent El Niño can trigger state transitions in global terrestrial ecosystems". Earth System Dynamics. 10 (4): 631–650. doi:10.5194/esd-10-631-2019. ISSN 2190-4979. S2CID 210348791.
- Schellnhuber, Hans Joachim; Rahmstorf, Stefan; Winkelmann, Ricarda (2016). "Why the right climate target was agreed in Paris". Nature Climate Change. 6 (7): 649–653. Bibcode:2016NatCC...6..649S. doi:10.1038/nclimate3013. ISSN 1758-6798.
- Arias, Paola A.; Bellouin, Nicolas; Coppola, Erika; Jones, Richard G.; et al. (2021). "Technical Summary" (PDF). IPCC AR6 WG1. p. 88.
- Stolbova, Veronika; Surovyatkina, Elena; Bookhagen, Bodo; Kurths, Jürgen (2016). "Tipping elements of the Indian monsoon: Prediction of onset and withdrawal". Geophysical Research Letters. 43 (8): 3982–3990. doi:10.1002/2016GL068392. S2CID 51811076.
- Katzenberger, Anja; Schewe, Jacob; Pongratz, Julia; Levermann, Anders (2021). "Robust increase of Indian monsoon rainfall and its variability under future warming in CMIP-6 models". Earth System Dynamics. 12 (2): 367–386. doi:10.5194/esd-12-367-2021.
- Arias, Paola A.; Bellouin, Nicolas; Coppola, Erika; Jones, Richard G.; et al. (2021). "Technical Summary" (PDF). IPCC AR6 WG1. p. 100.
- Schellnhuber, Hans Joachim; Winkelmann, Ricarda; Scheffer, Marten; Lade, Steven J.; Fetzer, Ingo; Donges, Jonathan F.; Crucifix, Michel; Cornell, Sarah E.; Barnosky, Anthony D. (2018). "Trajectories of the Earth System in the Anthropocene". Proceedings of the National Academy of Sciences. 115 (33): 8252–8259. Bibcode:2018PNAS..115.8252S. doi:10.1073/pnas.1810141115. ISSN 0027-8424. PMC 6099852. PMID 30082409.
- Sparrow, Katy J.; Kessler, John D.; Southon, John R.; Garcia-Tigreros, Fenix; Schreiner, Kathryn M.; Ruppel, Carolyn D.; Miller, John B.; Lehman, Scott J.; Xu, Xiaomei (17 January 2018). "Limited contribution of ancient methane to surface waters of the U.S. Beaufort Sea shelf". Science Advances. 4 (1). doi:10.1126/sciadv.aao4842.
- Mau, S.; Römer, M.; Torres, M. E.; Bussmann, I.; Pape, T.; Damm, E.; Geprägs, P.; Wintersteller, P.; Hsu, C.-W.; Loher, M.; Bohrmann, G. (23 February 2017). "Widespread methane seepage along the continental margin off Svalbard - from Bjørnøya to Kongsfjorden". Scientific Reports. 7. doi:10.1038/srep42997.
- Silyakova, Anna; Jansson, Pär; Serov, Pavel; Ferré, Benedicte; Pavlov, Alexey K.; Hattermann, Tore; Graves, Carolyn A.; Platt, Stephen M.; Lund Myhre, Cathrine; Gründger, Friederike; Niemann, Helge (1 February 2020). "Physical controls of dynamics of methane venting from a shallow seep area west of Svalbard". Continental Shelf Research. 194. doi:10.1016/j.csr.2019.104030.
- Fox-Kemper, B.; Hewitt, H.T.; Xiao, C.; Aðalgeirsdóttir, G.; Drijfhout, S.S.; Edwards, T.L.; Golledge, N.R.; Hemer, M.; Kopp, R.E.; Krinner, G.; Mix, A. (2021). Masson-Delmotte, V.; Zhai, P.; Pirani, A.; Connors, S.L.; Péan, C.; Berger, S.; Caud, N.; Chen, Y.; Goldfarb, L. (eds.). "Chapter 5: Global Carbon and other Biogeochemical Cycles and Feedbacks" (PDF). Climate Change 2021: The Physical Science Basis. Contribution of Working Group I to the Sixth Assessment Report of the Intergovernmental Panel on Climate Change. Cambridge University Press, Cambridge, UK and New York, NY, USA: 5. doi:10.1017/9781009157896.011 (inactive 9 July 2022).
{{cite journal}}
: CS1 maint: DOI inactive as of July 2022 (link) - Ashwin, Peter; Wieczorek, Sebastian; Vitolo, Renato; Cox, Peter (13 March 2012). "Tipping points in open systems: bifurcation, noise-induced and rate-dependent examples in the climate system". Philosophical Transactions of the Royal Society A: Mathematical, Physical and Engineering Sciences. 370 (1962): 1166–1184. arXiv:1103.0169. Bibcode:2012RSPTA.370.1166A. doi:10.1098/rsta.2011.0306. ISSN 1364-503X. PMID 22291228. S2CID 2324694.
- Rietkerk, Max; Bastiaansen, Robbin; Banerjee, Swarnendu; van de Koppel, Johan; Baudena, Mara; Doelman, Arjen (8 October 2021). "Evasion of tipping in complex systems through spatial pattern formation". Science. 374 (6564): eabj0359. doi:10.1126/science.abj0359. ISSN 0036-8075. PMID 34618584. S2CID 238476226.
- O'Keeffe, Paul E.; Wieczorek, Sebastian (1 January 2020). "Tipping Phenomena and Points of No Return in Ecosystems: Beyond Classical Bifurcations". SIAM Journal on Applied Dynamical Systems. 19 (4): 2371–2402. arXiv:1902.01796v7. doi:10.1137/19M1242884. S2CID 119316104.
- Boulton, Chris A.; Allison, Lesley C.; Lenton, Tim (December 2014). "Early warning signals of Atlantic Meridional Overturning Circulation collapse in a fully coupled climate model". Nature Communications. 5 (1): 5752. Bibcode:2014NatCo...5.5752B. doi:10.1038/ncomms6752. ISSN 2041-1723. PMC 4268699. PMID 25482065.
- Bathiany, Sebastian; Dijkstra, Henk; Crucifix, Michel; Dakos, Vasilis; Brovkin, Victor; Williamson, Mark S.; Lenton, Tim; Scheffer, Marten (2016). "Beyond bifurcation: using complex models to understand and predict abrupt climate change". Dynamics and Statistics of the Climate System. 1 (1): dzw004. doi:10.1093/climsys/dzw004. ISSN 2059-6987.
- Smith, Adam B.; Revilla, Eloy; Mindell, David P.; Matzke, Nicholas; Marshall, Charles; Kitzes, Justin; Gillespie, Rosemary; Williams, John W.; Vermeij, Geerat (2012). "Approaching a state shift in Earth's biosphere". Nature. 486 (7401): 52–58. Bibcode:2012Natur.486...52B. doi:10.1038/nature11018. hdl:10261/55208. ISSN 1476-4687. PMID 22678279. S2CID 4788164.
- Pollard, David; DeConto, Robert M. (2005). "Hysteresis in Cenozoic Antarctic ice-sheet variations". Global and Planetary Change. 45 (1–3): 9–12. Bibcode:2005GPC....45....9P. doi:10.1016/j.gloplacha.2004.09.011.
- Thomas, Zoë A. (15 November 2016). "Using natural archives to detect climate and environmental tipping points in the Earth System". Quaternary Science Reviews. 152: 60–71. Bibcode:2016QSRv..152...60T. doi:10.1016/j.quascirev.2016.09.026. ISSN 0277-3791. Archived from the original on 21 November 2021. Retrieved 20 April 2020.
- Lenton, Tim; Livina, V.N.; Dakos, V.; Van Nes, E.H.; Scheffer, M. (2012). "Early warning of climate tipping points from critical slowing down: comparing methods to improve robustness". Philosophical Transactions of the Royal Society A: Mathematical, Physical and Engineering Sciences. 370 (1962): 1185–1204. Bibcode:2012RSPTA.370.1185L. doi:10.1098/rsta.2011.0304. ISSN 1364-503X. PMC 3261433. PMID 22291229.
- Rosier, Sebastian (6 April 2021). "Guest post: Identifying three "tipping points" in Antarctica's Pine Island glacier". Carbon Brief. Archived from the original on 31 July 2021. Retrieved 1 August 2021.
- Rosier, Sebastian H. R.; Reese, Ronja; Donges, Jonathan F.; De Rydt, Jan; Gudmundsson, G. Hilmar; Winkelmann, Ricarda (25 March 2021). "The tipping points and early warning indicators for Pine Island Glacier, West Antarctica". The Cryosphere. 15 (3): 1501–1516. Bibcode:2021TCry...15.1501R. doi:10.5194/tc-15-1501-2021. ISSN 1994-0416. S2CID 233738686. Archived from the original on 1 August 2021. Retrieved 1 August 2021.
- Brovkin, Victor; Brook, Edward; Williams, John W.; Bathiany, Sebastian; et al. (29 July 2021). "Past abrupt changes, tipping points and cascading impacts in the Earth system". Nature Geoscience. 14 (8): 550–558. Bibcode:2021NatGe..14..550B. doi:10.1038/s41561-021-00790-5. S2CID 236504982. Archived from the original on 30 July 2021. Retrieved 1 August 2021.
- Liu, Yanlan; Kumar, Mukesh; Katul, Gabriel G.; Porporato, Amilcare (November 2019). "Reduced resilience as an early warning signal of forest mortality". Nature Climate Change. 9 (11): 880–885. Bibcode:2019NatCC...9..880L. doi:10.1038/s41558-019-0583-9. ISSN 1758-6798. S2CID 203848411. Archived from the original on 1 August 2021. Retrieved 1 August 2021.
- Boers, Niklas; Rypdal, Martin (25 May 2021). "Critical slowing down suggests that the western Greenland Ice Sheet is close to a tipping point". Proceedings of the National Academy of Sciences. 118 (21): e2024192118. Bibcode:2021PNAS..11824192B. doi:10.1073/pnas.2024192118. ISSN 0027-8424. PMC 8166178. PMID 34001613.
- Chen, D.; Rojas, M.; Samset, B.H.; Cobb, K.; et al. (2021). "Chapter 1: Framing, context, and methods" (PDF). In Masson-Delmotte, V. (ed.). Climate Change 2021: The Physical Science Basis. Contribution of Working Group I to the Sixth Assessment Report of the Intergovernmental Panel on Climate Change. Section 1.4.4.3.
- Lenton, Tim (2011). "Early warning of climate tipping points". Nature Climate Change. 1 (4): 201–209. CiteSeerX 10.1.1.666.244. doi:10.1038/nclimate1143. ISSN 1758-6798.
- Ditlevsen, Peter D.; Johnsen, Sigfus J. (2010). "Tipping points: Early warning and wishful thinking". Geophysical Research Letters. 37 (19): n/a. Bibcode:2010GeoRL..3719703D. doi:10.1029/2010GL044486. ISSN 1944-8007.
- Wieczorek, S.; Ashwin, P.; Luke, C. M.; Cox, P. M. (8 May 2011). "Excitability in ramped systems: the compost-bomb instability". Proceedings of the Royal Society A: Mathematical, Physical and Engineering Sciences. 467 (2129): 1243–1269. Bibcode:2011RSPSA.467.1243W. doi:10.1098/rspa.2010.0485. ISSN 1364-5021.
- Luke, C. M.; Cox, P. M. (2011). "Soil carbon and climate change: from the Jenkinson effect to the compost-bomb instability". European Journal of Soil Science. 62 (1): 5–12. doi:10.1111/j.1365-2389.2010.01312.x. ISSN 1365-2389. S2CID 55462001. Archived from the original on 21 November 2021. Retrieved 30 November 2019.
- Lohmann, Johannes; Ditlevsen, Peter D. (2021). "Risk of tipping the overturning circulation due to increasing rates of ice melt". Proceedings of the National Academy of Sciences. 118 (9): e2017989118. doi:10.1073/pnas.2017989118. ISSN 0027-8424. PMC 7936283. PMID 33619095.
- Rocha, Juan C.; Peterson, Garry; Bodin, Örjan; Levin, Simon (2018). "Cascading regime shifts within and across scales". Science. 362 (6421): 1379–1383. Bibcode:2018Sci...362.1379R. doi:10.1126/science.aat7850. ISSN 0036-8075. PMID 30573623. S2CID 56582186.
- "The irreversible emissions of a permafrost "tipping point"". World Economic Forum. Retrieved 17 July 2022.
- Wunderling, Nico; Donges, Jonathan F.; Kurths, Jürgen; Winkelmann, Ricarda (3 June 2021). "Interacting tipping elements increase risk of climate domino effects under global warming". Earth System Dynamics. 12 (2): 601–619. doi:10.5194/esd-12-601-2021. ISSN 2190-4979.
- Turner, Ben (12 June 2021). "Dramatic climate domino effects could be unleashed after less than 2 degrees of warming, a new study reveals". livescience.com. Retrieved 23 July 2022.
- Carrington, Damian (27 November 2019). "Climate emergency: world "may have crossed tipping points"". the Guardian.
- Dietz, Simon; Rising, James; Stoerk, Thomas; Wagner, Gernot (24 August 2021). "Economic impacts of tipping points in the climate system". Proceedings of the National Academy of Sciences. 118 (34): e2103081118. Bibcode:2021PNAS..11803081D. doi:10.1073/pnas.2103081118. PMC 8403967. PMID 34400500.
- Keen, Steve; Lenton, Timothy M.; Garrett, Timothy J.; Rae, James W. B.; Hanley, Brian P.; Grasselli, Matheus (19 May 2022). "Estimates of economic and environmental damages from tipping points cannot be reconciled with the scientific literature". Proceedings of the National Academy of Sciences. 119 (21): e2117308119. doi:10.1073/pnas.2117308119.
- Dietz, Simon; Rising, James; Stoerk, Thomas; Wagner, Gernot (19 May 2022). "Reply to Keen et al.: Dietz et al. modeling of climate tipping points is informative even if estimates are a probable lower bound". Proceedings of the National Academy of Sciences. 119 (21): e2201191119. doi:10.1073/pnas.2201191119.
- "What can Venus tell us about climate change on Earth?". BBC Sky at Night Magazine. Retrieved 18 July 2022.
- Dunbar, Brian (6 May 2015). "Venus". NASA. Retrieved 18 July 2022.
- Scoping of the IPCC 5th Assessment Report Cross Cutting Issues (PDF). Thirty-first Session of the IPCC Bali, 26–29 October 2009 (Report). Archived (PDF) from the original on 9 November 2009. Retrieved 24 March 2019.
- Hansen, James; Sato, Makiko; Russell, Gary; Kharecha, Pushker (2013). "Climate sensitivity, sea level and atmospheric carbon dioxide". Philosophical Transactions of the Royal Society A: Mathematical, Physical and Engineering Sciences. 371 (2001). 20120294. arXiv:1211.4846. Bibcode:2013RSPTA.37120294H. doi:10.1098/rsta.2012.0294. PMC 3785813. PMID 24043864.
External links
- Global Systems Institute Tipping points research at Exeter University
- The climate tipping points 2022 BBC radio documentary
