Hydrogen economy
The hydrogen economy is an umbrella term that draws together the roles hydrogen can play alongside renewable electricity to decarbonize specific economic sectors, sub-sectors and activities which may be technically difficult to decarbonize through other means, or where cheaper and more energy-efficient clean solutions are not available.[2] In this context, hydrogen economy encompasses hydrogen’s production through to end-uses in ways that substantively contribute to avoiding the use of fossil fuels and mitigating greenhouse gas emissions.

Most hydrogen produced today is ‘gray’ hydrogen, made from natural gas through steam methane reforming (SMR) which accounted for 1.8% of global greenhouse gas emissions in 2021.[3] Low-carbon hydrogen, which is made using SMR with carbon capture and storage ('blue' hydrogen), or through electrolysis of water using renewable power ('green' hydrogen), accounted for under 1% of production.[4] Virtually all hydrogen produced is used in oil refining (43% in 2021) and industry (57%), principally in the manufacture of ammonia for fertilizers, and methanol.[5]: 18, 22, 29
In its contribution to limiting global warming to 1.5°C, it is broadly envisaged that the future hydrogen economy replaces gray hydrogen with blue and predominantly green hydrogen, produced in greater total volumes, to provide for an expanded set of end-uses.[6] These are likely to be in heavy industry (e.g. high temperature processes alongside electricity, feedstock for production of green ammonia and organic chemicals, as alternative to coal-derived coke for steelmaking), long-haul transport (e.g. shipping, aviation and to a lesser extent heavy goods vehicles), and long-term energy storage.[7][8] Other applications, such as light duty vehicles and heating in buildings, are increasingly found to be out of scope for the future hydrogen economy, primarily for economic and environmental reasons.[9][10] These reasons include the difficulty of developing long-term storage, pipelines, and engine equipment, safety concerns since hydrogen is highly explosive, and the inefficiency of hydrogen compared to direct use of electricity.
The extent to which hydrogen will be used to decarbonise appropriate applications in heavy industry, long haul transport and long-term energy storage is likely to be influenced by the evolving production costs of low- and zero-carbon hydrogen. Estimates of future costs face numerous uncertainties – such as the introduction of carbon taxes, geography and geopolitics of energy, energy prices, technology choices, and their raw material requirements – but it is likely that green hydrogen will see the greatest reductions in production cost over time.[11]
History and contemporary rationale
Origins
The concept of the hydrogen economy, though not the term, was by geneticist J.B.S. Haldane in 1923, who, anticipating the exhaustion of Britain’s coal reserves for power generation, proposed a network of wind turbines to produce hydrogen for long-term energy storage through electrolysis, to help address renewable power’s variable output.[12] The term itself was coined by John Bockris during a talk he gave in 1970 at General Motors (GM) Technical Center.[13] Bockris viewed it as an economy in which hydrogen, underpinned by nuclear and solar power, would help address growing concern about fossil fuel depletion and environmental pollution, by serving as energy carrier for end-uses in which electrification was not suitable.[2]
A hydrogen economy was proposed by the University of Michigan to solve some of the negative effects of using hydrocarbon fuels where the carbon is released to the atmosphere (as carbon dioxide, carbon monoxide, unburnt hydrocarbons, etc.). Modern interest in the hydrogen economy can generally be traced to a 1970 technical report by Lawrence W. Jones of the University of Michigan,[14] in which he echoed Bockris’ dual rationale of addressing energy security and environmental challenges. Unlike Haldane and Bockris, Jones only focused on nuclear power as the energy source for electrolysis, and principally on the use of hydrogen in transport, where he regarded aviation and heavy goods transport as the top priorities.[15]
Later evolution

A spike in attention for the hydrogen economy concept during the 2000s was repeatedly described as hype by some critics and proponents of alternative technologies,[16][17][18] and investors lost money in the bubble.[19] Interest in the energy carrier resurged in the 2010s, notably with the forming of the World Hydrogen Council in 2017. Several manufacturers released hydrogen fuel cell cars commercially, with manufacturers such as Toyota, Hyundai, and industry groups in China having planned to increase numbers of the cars into the hundreds of thousands over the next decade.[20][21]
The global scope for hydrogen’s role in cars is shrinking relative to earlier expectations.[22][23] By the end of 2022, 70,200 hydrogen vehicles had been sold worldwide,[24] compared with 26 million plug-in electric vehicles.[25]
Contemporary takes on the hydrogen economy share earlier perspectives’ emphasis on the complementarity of electricity and hydrogen, and the use of electrolysis as the mainstay of hydrogen production.[26] They focus on the need to limit global warming to 1.5C and prioritise the production, transportation and use of green hydrogen for heavy industry (e.g. high-temperature processes alongside electricity,[27] feedstock for production of green ammonia and organic chemicals,[28] as alternative to coal-derived coke for steelmaking),[29] long-haul transport (e.g. shipping, aviation and to a lesser extent heavy goods vehicles), and long-term energy storage.[26][30]
Current hydrogen market
Hydrogen production globally was valued at over US$155 billion in 2022 and is expected to grow over 9% annually through 2030.[31]
In 2021, 94 million tonnes (Mt) of molecular hydrogen (H2) was produced.[32] Of this total, approximately one sixth was as a by-product of petrochemical industry processes.[33] Most hydrogen comes from dedicated production facilities, over 99% of which is from fossil fuels, mainly via steam reforming of natural gas (70%) and coal gasification (30%, almost all of which in China).[33] Less than 1% of dedicated hydrogen production is low carbon: steam fossil fuel reforming with carbon capture and storage, green hydrogen produced using electrolysis, and hydrogen produced from biomass.[33] CO2 emissions from 2021 production, at 915 MtCO2,[34] amounted to 2.5% of energy-related CO2 emissions[35] and 1.8% of global greenhouse gas emissions.[36]
Virtually all hydrogen produced for the current market is used in oil refining (40 MtH2 in 2021) and industry (54 MtH2).[37]: 18, 22 In oil refining, hydrogen is used, in a process known as hydrocracking, to convert heavy petroleum sources into lighter fractions suitable for use as fuels. Industrial uses mainly comprise ammonia production to make fertilisers (34 MtH2 in 2021), methanol production (15 MtH2) and the manufacture of direct reduced iron (5 MtH2).[37]: 29
Production
As of 2022, more than 95% of global hydrogen production is sourced from fossil gas and coal without carbon abatement.[38]: 1
Color codes
Hydrogen is often referred to by various colors to indicate its origin (perhaps because gray symbolizes "dirty hydrogen"[19]).[39]
Color | Production source | Notes | References | |
---|---|---|---|---|
green | renewable electricity | via electrolysis of water | [41]: 28 | |
turquoise | thermal splitting of methane | via methane pyrolysis | [41]: 28 [42]: 2 | |
blue | hydrocarbons with carbon capture and storage | CCS networks required | [41]: 28 | |
gray | fossil hydrocarbons, mainly steam reforming of natural gas | [41]: 28 [43]: 10 [42]: 2 | ||
brown or black | fossil hydrocarbons: brown (lignite) or black coal | [44]: 91 | ||
pink or purple | nuclear power | via electrolysis of water, or contributing steam to natural gas reforming | [42]: 2 [19] | |
yellow | sometimes understood to mean solar photovoltaics, or a mix of renewable and fossil electricity | via photovoltaic | [39] | |
gold or white | hydrogen that occurs naturally deep within the Earth's crust | obtained by mining; also referred to as white | [45] |
Methods of production
Molecular hydrogen was discovered in the Kola Superdeep Borehole. It is unclear how much molecular hydrogen is available in natural reservoirs, but at least one company[46] specializes in drilling wells to extract hydrogen. Most hydrogen in the lithosphere is bonded to oxygen in water. Manufacturing elemental hydrogen requires the consumption of a hydrogen carrier such as a fossil fuel or water. The former carrier consumes the fossil resource and in the steam methane reforming (SMR) process produces greenhouse gas carbon dioxide. However, in the newer methane pyrolysis process no greenhouse gas carbon dioxide is produced. These processes typically require no further energy input beyond the fossil fuel.

Decomposing water, the latter carrier, requires electrical or heat input, generated from some primary energy source (fossil fuel, nuclear power or a renewable energy). Hydrogen produced by electrolysis of water using renewable energy sources such as wind and solar power, referred to as green hydrogen.[47] When derived from natural gas by zero greenhouse emission methane pyrolysis, it is referred to as turquoise hydrogen.[48]
When fossil fuel derived with greenhouse gas emissions, is generally referred to as grey hydrogen. If most of the carbon dioxide emission is captured, it is referred to as blue hydrogen.[49] Hydrogen produced from coal may be referred to as brown or black hydrogen.[50]
Steam reforming – gray or blue
Hydrogen is industrially produced from steam reforming (SMR), which uses natural gas.[51] The energy content of the produced hydrogen is around 74% of the energy content of the original fuel,[52] as some energy is lost as excess heat during production. In general, steam reforming emits carbon dioxide, a greenhouse gas, and is known as gray hydrogen. If the carbon dioxide is captured and stored, the hydrogen produced is known as blue hydrogen.
Electrolysis of water – green, pink or yellow
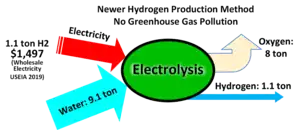
Hydrogen can be made via high pressure electrolysis, low pressure electrolysis of water, or a range of other emerging electrochemical processes such as high temperature electrolysis or carbon assisted electrolysis.[53] However, current best processes for water electrolysis have an effective electrical efficiency of 70-80%,[54][55][56] so that producing 1 kg of hydrogen (which has a specific energy of 143 MJ/kg or about 40 kWh/kg) requires 50–55 kWh of electricity.
In parts of the world, steam methane reforming is between $1–3/kg on average excluding hydrogen gas pressurization cost. This makes production of hydrogen via electrolysis cost competitive in many regions already, as outlined by Nel Hydrogen[57] and others, including an article by the IEA[58] examining the conditions which could lead to a competitive advantage for electrolysis.
A small part (2% in 2019[59]) is produced by electrolysis using electricity and water, consuming approximately 50 to 55 kilowatt-hours of electricity per kilogram of hydrogen produced.[60]
Hydrogen as a byproduct of other chemical processes
The industrial production of chlorine and caustic soda by electrolysis generates a sizable amount of Hydrogen as a byproduct. In the port of Antwerp a 1MW demonstration fuel cell power plant is powered by such byproduct. This unit has been operational since late 2011.[61] The excess hydrogen is often managed with a hydrogen pinch analysis.
Gas generated from coke ovens in steel production is similar to Syngas with 60% hydrogen by volume.[62] The hydrogen can be extracted from the coke oven gas economically.[63]
Use as an energy carrier

Hydrogen can be deployed as a fuel in two distinct ways: in fuel cells which produce electricity, and via combustion to generate heat.[64] When hydrogen is consumed in fuel cells, the only emission at the point of use is water vapour.[64] Combustion of hydrogen can lead to the thermal formation of harmful nitrogen oxides emissions.[64]
Industry
In the context of limiting global warming, low-carbon hydrogen (particularly green hydrogen) is likely to play an important role in decarbonising industry.[65] Hydrogen fuel can produce the intense heat required for industrial production of steel, cement, glass, and chemicals, thus contributing to the decarbonisation of industry alongside other technologies, such as electric arc furnaces for steelmaking.[66] However, it is likely to play a larger role in providing industrial feedstock for cleaner production of ammonia and organic chemicals.[65] For example, in steelmaking, hydrogen could function as a clean energy carrier and also as a low-carbon catalyst replacing coal-derived coke.[67]
The imperative to use low-carbon hydrogen to reduce greenhouse gas emissions has the potential to reshape the geography of industrial activities, as locations with appropriate hydrogen production potential in different regions will interact in new ways with logistics infrastructure, raw material availability, human and technological capital.[65]
Transport
Much of the interest in the hydrogen economy concept is focused on the use of fuel cells to power hydrogen vehicles, particularly large trucks. Hydrogen vehicles produce significantly less local air pollution than conventional vehicles.[68] By 2050, the energy requirement for transportation might be between 20% and 30% fulfilled by hydrogen and synthetic fuels.[69][70][71]
Hydrogen used to decarbonise transportation is likely to find its largest applications in shipping, aviation and to a lesser extent heavy goods vehicles, through the use of hydrogen-derived synthetic fuels such as ammonia and methanol, and fuel cell technology.[72] Hydrogen has been used in fuel cell buses for many years. It is also used as a fuel for spacecraft propulsion.
In the light road vehicle segment including passenger cars, by the end of 2022, 70,200 fuel cell electric vehicles had been sold worldwide,[73] compared with 26 million plug-in electric vehicles.[74] With the rapid rise of electric vehicles and associated battery technology and infrastructure, the global scope for hydrogen’s role in cars is shrinking relative to earlier expectations.[75][76]
In the International Energy Agency’s 2022 Net Zero Emissions Scenario (NZE), hydrogen is forecast to account for 2% of rail energy demand in 2050, while 90% of rail travel is expected to be electrified by then (up from 45% today). Hydrogen’s role in rail would likely be focused on lines that prove difficult or costly to electrify.[77] The NZE foresees hydrogen meeting approximately 30% of heavy truck energy demand in 2050, mainly for long-distance heavy freight (with battery electric power accounting for around 60%).[78]
Although hydrogen can be used in adapted internal combustion engines, fuel cells, being electrochemical, have an efficiency advantage over heat engines. Fuel cells are more expensive to produce than common internal combustion engines.
Buildings
Numerous industry groups (gas networks, gas boiler manufacturers) across the natural gas supply chain are promoting hydrogen combustion boilers for space and water heating, and hydrogen appliances for cooking, to reduce energy-related CO2 emissions from residential and commercial buildings.[79][80][81] The proposition is that current end-users of piped natural gas can await the conversion of and supply of hydrogen to existing natural gas grids, and then swap heating and cooking appliances, and that there is no need for consumers to do anything now.[79][80][81]
A review of 32 studies on the question of hydrogen for heating buildings, independent of commercial interests, found that the economics and climate benefits of hydrogen for heating and cooking generally compare very poorly with the deployment of district heating networks, electrification of heating (principally through heat pumps) and cooking, the use of solar thermal, waste heat and the installation of energy efficiency measures to reduce energy demand for heat.[81] Due to inefficiencies in hydrogen production, using blue hydrogen to replace natural gas for heating could require three times as much methane, while using green hydrogen would need two to three times as much electricity as heat pumps.[81] Hybrid heat pumps, which combine the use of an electric heat pump with a hydrogen boiler, may play a role in residential heating in areas where upgrading networks to meet peak electrical demand would otherwise be costly.[81]
The widespread use of hydrogen for heating buildings would entail higher energy system costs, higher heating costs and higher environmental impacts than the alternatives, although a niche role may be appropriate in specific contexts and geographies.[81] If deployed, using hydrogen in buildings would drive up the cost of hydrogen for harder-to-decarbonise applications in industry and transport.[81]
Energy system balancing and storage
Green hydrogen, from electrolysis of water, has the potential to address the variability of renewable energy output. Producing green hydrogen can both reduce the need for renewable power curtailment during periods of high renewables output and be stored long-term to provide for power generation during periods of low output.[82][83]
Ammonia
An alternative to gaseous hydrogen as an energy carrier is to bond it with nitrogen from the air to produce ammonia, which can be easily liquefied, transported, and used (directly or indirectly) as a clean and renewable fuel.[84][85] Among disadvantages of ammonia as an energy carrier are its high toxicity, extremely low energy efficiency of NH3 production from N2 and H2, and poisoning of PEM Fuel Cells by traces of non-decomposed NH3 after NH3 to N2 conversion.
Bio-SNG
As of 2019 although technically possible production of syngas from hydrogen and carbon-dioxide from bio-energy with carbon capture and storage (BECCS) via the Sabatier reaction is limited by the amount of sustainable bioenergy available:[86] therefore any bio-SNG made may be reserved for production of aviation biofuel.[87]
Storage
Although molecular hydrogen has very high energy density on a mass basis, partly because of its low molecular weight, as a gas at ambient conditions it has very low energy density by volume. If it is to be used as fuel stored on board the vehicle, pure hydrogen gas must be stored in an energy-dense form to provide sufficient driving range. Because hydrogen is the smallest molecule, it easily escapes from containers, and leaked hydrogen has a global warming effect 11.6 times stronger than CO₂.[88]
Pressurized hydrogen gas
Increasing gas pressure improves the energy density by volume making for smaller container tanks. The standard material for holding pressurised hydrogen in tube trailers is steel (there is no hydrogen embrittlement problem with hydrogen gas). Tanks made of carbon and glass fibres reinforcing plastic as fitted in Toyota Marai and Kenworth trucks are required to meet safety standards. Few materials are suitable for tanks as hydrogen being a small molecule tends to diffuse through many polymeric materials. The most common on board hydrogen storage in today's 2020 vehicles is hydrogen at pressure 700bar = 70MPa. The energy cost of compressing hydrogen to this pressure is significant.
Pressurized gas pipelines are always made of steel and operate at much lower pressures than tube trailers.
Liquid hydrogen
Alternatively, higher volumetric energy density liquid hydrogen or slush hydrogen may be used. However, liquid hydrogen is cryogenic and boils at 20.268 K (–252.882 °C or –423.188 °F). Cryogenic storage cuts weight but requires large liquification energies. The liquefaction process, involving pressurizing and cooling steps, is energy intensive.[89] The liquefied hydrogen has lower energy density by volume than gasoline by approximately a factor of four, because of the low density of liquid hydrogen – there are actually more oxidizable hydrogen atoms in a litre of gasoline (116 grams) than there are in a litre of pure liquid hydrogen (71 grams). Like any other liquid at cryogenic temperatures, the liquid hydrogen storage tanks must also be well insulated to minimize boil off.
Japan has a liquid hydrogen (LH2) storage facility at a terminal in Kobe, and was expected to receive the first shipment of liquid hydrogen via LH2 carrier in 2020.[90] Hydrogen is liquified by reducing its temperature to -253 °C, similar to liquified natural gas (LNG) which is stored at -162 °C. A potential efficiency loss of 12.79% can be achieved, or 4.26kWh/kg out of 33.3kWh/kg.[91]
Liquid organic hydrogen carriers (LOHC)
Storage as hydride
Distinct from storing molecular hydrogen, hydrogen can be stored as a chemical hydride or in some other hydrogen-containing compound. Hydrogen gas is reacted with some other materials to produce the hydrogen storage material, which can be transported relatively easily. At the point of use the hydrogen storage material can be made to decompose, yielding hydrogen gas. As well as the mass and volume density problems associated with molecular hydrogen storage, current barriers to practical storage schemes stem from the high pressure and temperature conditions needed for hydride formation and hydrogen release.
For many potential systems hydriding and dehydriding kinetics and heat management are also issues that need to be overcome. A French company McPhy Energy is developing the first industrial product, based on Magnesium Hydrate, already sold to some major clients such as Iwatani and ENEL. Emergent hydride hydrogen storage technologies have achieved a compressed volume of less than 1/500.
Adsorption
A third approach is to adsorb molecular hydrogen on the surface of a solid storage material. Unlike in the hydrides mentioned above, the hydrogen does not dissociate/recombine upon charging/discharging the storage system, and hence does not suffer from the kinetic limitations of many hydride storage systems. Hydrogen densities similar to liquefied hydrogen can be achieved with appropriate adsorbent materials. Some suggested adsorbents include activated carbon, nanostructured carbons (including CNTs), MOFs, and hydrogen clathrate hydrate.
Underground hydrogen storage

Underground hydrogen storage is the practice of hydrogen storage in caverns, salt domes and depleted oil and gas fields. Large quantities of gaseous hydrogen have been stored in caverns by ICI for many years without any difficulties.[92] The storage of large quantities of liquid hydrogen underground can function as grid energy storage. The round-trip efficiency is approximately 40% (vs. 75-80% for pumped-hydro (PHES)), and the cost is slightly higher than pumped hydro.[93]
Another study referenced by a European staff working paper found that for large scale storage, the cheapest option is hydrogen at €140/MWh for 2,000 hours of storage using an electrolyser, salt cavern storage and combined-cycle power plant.[94] The European project Hyunder[95] indicated in 2013 that for the storage of wind and solar energy an additional 85 caverns are required as it cannot be covered by PHES and CAES systems.[96]
A German case study on storage of hydrogen in salt caverns found that if the German power surplus (7% of total variable renewable generation by 2025 and 20% by 2050) would be converted to hydrogen and stored underground, these quantities would require some 15 caverns of 500,000 cubic metres each by 2025 and some 60 caverns by 2050 – corresponding to approximately one third of the number of gas caverns currently operated in Germany.[97] In the US, Sandia Labs are conducting research into the storage of hydrogen in depleted oil and gas fields, which could easily absorb large amounts of renewably produced hydrogen as there are some 2.7 million depleted wells in existence.[98]
Infrastructure

The hydrogen infrastructure would consist mainly of industrial hydrogen pipeline transport and hydrogen-equipped filling stations like those found on a hydrogen highway. Hydrogen stations which were not situated near a hydrogen pipeline would get supply via hydrogen tanks, compressed hydrogen tube trailers, liquid hydrogen trailers, liquid hydrogen tank trucks or dedicated onsite production.
Over 700 miles of hydrogen pipeline currently exist in the United States. Although expensive, pipelines are the cheapest way to move hydrogen over long distances. Hydrogen gas piping is routine in large oil-refineries, because hydrogen is used to hydrocrack fuels from crude oil.
Hydrogen embrittlement is not a problem for hydrogen gas pipelines. Hydrogen embrittlement only happens with 'diffusible' hydrogen, i.e. atoms or ions. Hydrogen gas, however, is molecular (H2), and there is a very significant energy barrier to splitting it into atoms.[99]
The IEA recommends existing industrial ports be used for production and existing natural gas pipelines for transport: also international co-operation and shipping.[100]
South Korea and Japan,[101] which as of 2019 lack international electrical interconnectors, are investing in the hydrogen economy.[102] In March 2020, the Fukushima Hydrogen Energy Research Field was opened in Japan, claiming to be the world's largest hydrogen production facility.[103] The site occupies 180,000 m2 (1,900,000 sq ft) of land, much of which is occupied by a solar array; power from the grid is also used for electrolysis of water to produce hydrogen fuel.[104]
A key tradeoff: centralized vs. distributed production
In a future full hydrogen economy, primary energy sources and feedstock would be used to produce hydrogen gas as stored energy for use in various sectors of the economy. Producing hydrogen from primary energy sources other than coal and oil would result in lower production of the greenhouse gases characteristic of the combustion of coal and oil fossil energy resources. The importance of non-polluting methane pyrolysis of natural gas is becoming a recognized method for using current natural gas infrastructure investment to produce hydrogen and no greenhouse gas.
One key feature of a hydrogen economy would be that in mobile applications (primarily vehicular transport) energy generation and use could be decoupled. The primary energy source would need no longer travel with the vehicle, as it currently does with hydrocarbon fuels. Instead of tailpipes creating dispersed emissions, the energy (and pollution) could be generated from point sources such as large-scale, centralized facilities with improved efficiency. This would allow the possibility of technologies such as carbon sequestration, which are otherwise impossible for mobile applications. Alternatively, distributed energy generation schemes (such as small scale renewable energy sources) could be used, possibly associated with hydrogen stations.
Aside from the energy generation, hydrogen production could be centralized, distributed or a mixture of both. While generating hydrogen at centralized primary energy plants promises higher hydrogen production efficiency, difficulties in high-volume, long range hydrogen transportation (due to factors such as hydrogen damage and the ease of hydrogen diffusion through solid materials) makes electrical energy distribution attractive within a hydrogen economy.
In such a scenario, small regional plants or even local filling stations could generate hydrogen using energy provided through the electrical distribution grid or methane pyrolysis of natural gas. While hydrogen generation efficiency is likely to be lower than for centralized hydrogen generation, losses in hydrogen transport could make such a scheme more efficient in terms of the primary energy used per kilogram of hydrogen delivered to the end user.
The proper balance between hydrogen distribution, long-distance electrical distribution and destination converted pyrolysis of natural gas is one of the primary questions that arises about the hydrogen economy.
Again the dilemmas of production sources and transportation of hydrogen can now be overcome using on site (home, business, or fuel station) generation of hydrogen from off grid renewable sources.
Distributed electrolysis
Distributed electrolysis would bypass the problems of distributing hydrogen by distributing electricity instead. It would use existing electrical networks to transport electricity to small, on-site electrolysers located at filling stations. However, accounting for the energy used to produce the electricity and transmission losses would reduce the overall efficiency.
Safety
Hydrogen has one of the widest explosive/ignition mix range with air of all the gases with few exceptions such as acetylene, silane, and ethylene oxide, and in terms of minimum necessary ignition energy and mixture ratios has extremely low requirements for an explosion to occur. This means that whatever the mix proportion between air and hydrogen, when ignited in an enclosed space a hydrogen leak will most likely lead to an explosion, not a mere flame.[105] Systems and procedures to avoid accidents involve considering:
- Inerting hydrogen lines
- Systems to quickly purge hydrogen or ventilate an area
- Flaring off hydrogen
- Ignition source management
- Taking into account mechanical integrity issues
- Identifying possible hydrogen gas as a byproduct in certain chemical reactions
- Detecting hydrogen leaks or flames
- Inventory management
- Properly spacing hydrogen and other flammable material
- Specialized pressurized hydrogen containment tanks, in particular with cryogenic hydrogen
- Other human factors
There are many codes and standards regarding hydrogen safety in storage, transport, and use. These range from federal regulations,[106] ANSI/AIAA,[107] NFPA,[108] and ISO[109] standards. The Canadian Hydrogen Safety Program concluded that hydrogen fueling is as safe as, or safer than, compressed natural gas (CNG) fueling,[110]
Costs
More widespread use of hydrogen in economies entails the need for investment and costs in its production, storage, distribution and use. Estimates of hydrogen’s cost are therefore complex and need to make assumptions about the cost of energy inputs (typically gas and electricity), production plant and method (e.g. green or blue hydrogen), technologies used (e.g. alkaline or proton exchange membrane electrolysers), storage and distribution methods, and how different cost elements might change over time.[111]: 49–65 These factors are incorporated into calculations of the levelized costs of hydrogen (LCOH). The following table shows a range of estimates of the levelized costs of gray, blue, and green hydrogen, expressed in terms of US$ per kg of H2.[note 1]
Production method | Note | Current cost (2020-2022) | Projected 2030 cost | Projected 2050 cost |
Gray hydrogen (not including a carbon tax) | ||||
International Energy Agency[112] | 2022 costs estimated for June, when gas prices peaked in the wake of Russia’s invasion of Ukraine | 2021: 1.0-2.5 | - | - |
2022: 4.8-7.8 | ||||
PWC[113] | 2021: 1.2-2.4 | |||
Blue hydrogen | ||||
International Energy Agency[112] | 2022 costs estimated for June, when gas prices peaked in the wake of Russia’s invasion of Ukraine | 2021: 1.5-3.0 | - | - |
2022: 5.3-8.6 | ||||
UK government[114] | Range dependent on gas price | 2020: 1.6-2.7 | 1.6-2.7 | 1.6-2.8 |
GEP[115] | 2022: 2.8-3.5 | - | - | |
Energy Transitions Commission[111]: 28 | 2020: 1.5-2.4 | 1.3-2.3 | 1.4-2.2 | |
Green hydrogen | ||||
International Energy Agency[112] | 2030 and 2050 estimates are using solar power in regions with good solar conditions | 2021: 4.0-9.0 | <1.5 | <1.0 |
2022: 3.0-4.3 | ||||
UK government[114] | Using grid electricity, UK specific; range dependent on electricity price, and electrolyser technology and cost | 2020: 4.9-7.9 | 4.4-6.6 | 4.0-6.3 |
Using otherwise curtailed renewable electricity, UK specific; range dependent on electrolyser technology and cost | 2020: 2.4-7.9 | 1.7-5.6 | 1.5-4.6 | |
IRENA[116] | 2020: 2.2-5.2 | 1.4-4.1 | 1.1-3.4 | |
GEP[115] | Source notes green H2 production cost has fallen by 60% since 2010 | 2022: 3.0-6.0 | ||
Lazard[117] | 2022: 2.8-5.3 | |||
PWC[113] | 2021: 3.5-9.5 | 1.8-4.8 | 1.2-2.4 | |
Energy Transitions Commission[111]: 28 | 2020: 2.6-3.6 | 1.0-1.7 | 0.7-1.2 |
The range of cost estimates for commercially available hydrogen production methods is broad, As of 2022, gray hydrogen is cheapest to produce without a tax on its CO2 emissions, followed by blue and green hydrogen. Blue hydrogen production costs are not anticipated to fall substantially by 2050,[114][111]: 28 can be expected to fluctuate with natural gas prices and could face carbon taxes for uncaptured emissions.[111]: 79 The cost of electrolysers fell by 60% from 2010 to 2022,[115] before rising slightly due to an increasing cost of capital.[19] Their cost is projected to fall significantly to 2030 and 2050,[118]: 26 driving down the cost of green hydrogen alongside the falling cost of renewable power generation.[119][111]: 28 It is cheapest to produce green hydrogen with surplus renewable power that would otherwise be curtailed, which favours electrolysers capable of responding to low and variable power levels.[118]: 5
A 2022 Goldman Sachs analysis anticipates that globally green hydrogen will achieve cost parity with grey hydrogen by 2030, earlier if a global carbon tax is placed on gray hydrogen.[120] In terms of cost per unit of energy, blue and gray hydrogen will always cost more than the fossil fuels used in its production, while green hydrogen will always cost more than the renewable electricity used to make it.
Subsidies for clean hydrogen production are much higher in the US and EU than in India.[121]
Examples and pilot programs

The distribution of hydrogen for the purpose of transportation is being tested around the world, particularly in the US (California, Massachusetts), Canada, Japan, the EU (Portugal, Norway, Denmark, Germany), and Iceland.
Several domestic U.S. automobile have developed vehicles using hydrogen, such as GM and Toyota.[122] However, as of February 2020, infrastructure for hydrogen was underdeveloped except in some parts of California.[123] The United States have their own hydrogen policy. A joint venture between NREL and Xcel Energy is combining wind power and hydrogen power in the same way in Colorado.[124] Hydro in Newfoundland and Labrador are converting the current wind-diesel Power System on the remote island of Ramea into a Wind-Hydrogen Hybrid Power Systems facility.[125]
A similar pilot project on Stuart Island uses solar power, instead of wind power, to generate electricity. When excess electricity is available after the batteries are fully charged, hydrogen is generated by electrolysis and stored for later production of electricity by fuel cell.[126] The US also have a large natural gas pipeline system already in place.[127]
Countries in the EU which have a relatively large natural gas pipeline system already in place include Belgium, Germany, France, and the Netherlands.[127] In 2020, The EU launched its European Clean Hydrogen Alliance (ECHA).[128][129]
The UK started a fuel cell pilot program in January 2004, the program ran two Fuel cell buses on route 25 in London until December 2005, and switched to route RV1 until January 2007.[130] The Hydrogen Expedition is currently working to create a hydrogen fuel cell-powered ship and using it to circumnavigate the globe, as a way to demonstrate the capability of hydrogen fuel cells.[131] In August 2021 the UK Government claimed it was the first to have a Hydrogen Strategy and produced a document.[132]
Western Australia's Department of Planning and Infrastructure operated three Daimler Chrysler Citaro fuel cell buses as part of its Sustainable Transport Energy for Perth Fuel Cells Bus Trial in Perth.[133] The buses were operated by Path Transit on regular Transperth public bus routes. The trial began in September 2004 and concluded in September 2007. The buses' fuel cells used a proton exchange membrane system and were supplied with raw hydrogen from a BP refinery in Kwinana, south of Perth. The hydrogen was a byproduct of the refinery's industrial process. The buses were refueled at a station in the northern Perth suburb of Malaga.
Iceland has committed to becoming the world's first hydrogen economy by the year 2050.[134] Iceland is in a unique position. Presently, it imports all the petroleum products necessary to power its automobiles and fishing fleet. Iceland has large geothermal resources, so much that the local price of electricity actually is lower than the price of the hydrocarbons that could be used to produce that electricity.
Iceland already converts its surplus electricity into exportable goods and hydrocarbon replacements. In 2002, it produced 2,000 tons of hydrogen gas by electrolysis, primarily for the production of ammonia (NH3) for fertilizer. Ammonia is produced, transported, and used throughout the world, and 90% of the cost of ammonia is the cost of the energy to produce it.
Neither industry directly replaces hydrocarbons. Reykjavík, Iceland, had a small pilot fleet of city buses running on compressed hydrogen,[135] and research on powering the nation's fishing fleet with hydrogen is under way (for example by companies as Icelandic New Energy). For more practical purposes, Iceland might process imported oil with hydrogen to extend it, rather than to replace it altogether.
The Reykjavík buses are part of a larger program, HyFLEET:CUTE,[136] operating hydrogen fueled buses in eight European cities. HyFLEET:CUTE buses were also operated in Beijing, China and Perth, Australia (see below). A pilot project demonstrating a hydrogen economy is operational on the Norwegian island of Utsira. The installation combines wind power and hydrogen power. In periods when there is surplus wind energy, the excess power is used for generating hydrogen by electrolysis. The hydrogen is stored, and is available for power generation in periods when there is little wind.
India is said to adopt hydrogen and H-CNG, due to several reasons, amongst which the fact that a national rollout of natural gas networks is already taking place and natural gas is already a major vehicle fuel. In addition, India suffers from extreme air pollution in urban areas.[137][138] According to some estimates, nearly 80% of India's hydrogen is projected to be green, driven by cost declines and new production technologies.[139]
Currently however, hydrogen energy is just at the Research, Development and Demonstration (RD&D) stage.[140][141] As a result, the number of hydrogen stations may still be low,[142] although much more are expected to be introduced soon.[143][144][145]
The Turkish Ministry of Energy and Natural Resources and the United Nations Industrial Development Organization have signed a $40 million trust fund agreement in 2003 for the creation of the International Centre for Hydrogen Energy Technologies (UNIDO-ICHET) in Istanbul, which started operation in 2004.[146] A hydrogen forklift, a hydrogen cart and a mobile house powered by renewable energies are being demonstrated in UNIDO-ICHET's premises. An uninterruptible power supply system has been working since April 2009 in the headquarters of Istanbul Sea Buses company.
Another indicator of the presence of large natural gas infrastructures already in place in countries and in use by citizens is the number of natural gas vehicles present in the country. The countries with the largest amount of natural gas vehicles are (in order of magnitude):[147] Iran, China, Pakistan, Argentina, India, Brasil, Italy, Colombia, Thailand, Uzbekistan, Bolivia, Armenia, Bangladesh, Egypt, Peru, Ukraine, United States. Natural gas vehicles can also be converted to run on hydrogen.
Some hospitals have installed combined electrolyser-storage-fuel cell units for local emergency power. These are advantageous for emergency use because of their low maintenance requirement and ease of location compared to internal combustion driven generators.
Also, in some private homes, fuel cell micro-CHP plants can be found, which can operate on hydrogen, or other fuels as natural gas or LPG.[148][149] When running on natural gas, it relies on steam reforming of natural gas to convert the natural gas to hydrogen prior to use in the fuel cell. This hence still emits CO2 (see reaction) but (temporarily) running on this can be a good solution until the point where the hydrogen is starting to become distributed through the (natural gas) piping system.
In October 2021, Queensland Premier Annastacia Palaszczuk and Andrew Forrest announced that Queensland will be home to the world's largest hydrogen plant.[150]
German car manufacturer BMW has also been working with hydrogen for years.[151]
Saudi Arabia as a part of the NEOM project, is looking to produce roughly 1.2 million tonnes of green ammonia a year, beginning production in 2025.[152]
In Australia, the Australian Renewable Energy Agency (ARENA) has invested $55 million in 28 hydrogen projects, from early stage research and development to early stage trials and deployments. The agency's stated goal is to produce hydrogen by electrolysis for $2 per kilogram, announced by Minister for Energy and Emissions Angus Taylor in a 2021 Low Emissions Technology Statement.[153]
In August 2021, Chris Jackson quit as chair of the UK Hydrogen and Fuel Cell Association, a leading hydrogen industry association, claiming that UK and Norwegian oil companies had intentionally inflated their cost projections for blue hydrogen in order to maximize future technology support payments by the UK government.[154]
Green hydrogen has become more common in France. A €150 million Green Hydrogen Plan was established in 2019, and it calls for building the infrastructure necessary to create, store, and distribute hydrogen as well as using the fuel to power local transportation systems like buses and trains. Corridor H2, a similar initiative, will create a network of hydrogen distribution facilities in Occitania along the route between the Mediterranean and the North Sea. The Corridor H2 project will get a €40 million loan from the EIB.[155][156]
Timeline of results
- 2022
- Researchers increase water electrolysis performance of renewable hydrogen via capillary-fed electrolysis cells.[157][158]
- A novel energy-efficient strategy for hydrogen release from liquid hydrogen carriers with the potential to reduce costs of storage and transportation is reported.[159][160]
- Researchers report the development of a potential efficient, secure and convenient method to separate, purify, store and transport large amounts of hydrogen for energy storage in renewables-based energy systems as powder using ball milling.[161][162]
- A way method for hydrogen production from the air, useful for off-the-grid settings, is demonstrated.[163][164]
- A novel type of effective hydrogen storage using readily available salts is reported.[165][166]
- An electrolysis system for viable hydrogen production from seawater without requiring a pre-desalination process is reported, which could allow for more flexible and less costly hydrogen production.[167][168]
- Chemical engineers report a method to substantially increase conversion efficiency and reduce material costs of green hydrogen production by using sound waves during electrolysis.[169][170]
- 2023
- In three largely paywalled studies, separate teams of researchers report substantial improvements to green hydrogen production methods, enabling higher efficiencies and durable use of untreated seawater.[171][172][173][174][175][176]
- A DVGW report suggests gas pipeline infrastructures (in Germany) are suitable to be repurposed to transport hydrogen, showing limited corrosion.[177][178]
- A concentrated solar-to-hydrogen device approaching viability is demonstrated.[179][180]
- Record solar-to-hydrogen efficiencies, using photoelectrochemical cells, are reported.[181]
Experimental production methods
Methane pyrolysis – turquoise

Pyrolysis of methane (natural gas) with a one-step process[182] bubbling methane through a molten metal catalyst is a "no greenhouse gas" approach to produce hydrogen that was demonstrated in laboratory conditions in 2017 and now being tested at larger scales.[183][48] The process is conducted at high temperatures (1065 °C).[184][185][186][187] Producing 1 kg of hydrogen requires about 18 kWh of electricity for process heat.[188] The pyrolysis of methane can be expressed by the following reaction equation.[189]
- CH
4(g) → C(s) + 2 H
2(g) ΔH° = 74.8 kJ/mol
The industrial quality solid carbon may be sold as manufacturing feedstock or landfilled (no pollution).
Biological production
Fermentative hydrogen production is the fermentative conversion of organic substrate to biohydrogen manifested by a diverse group of bacteria using multi enzyme systems involving three steps similar to anaerobic conversion. Dark fermentation reactions do not require light energy, so they are capable of constantly producing hydrogen from organic compounds throughout the day and night. Photofermentation differs from dark fermentation because it only proceeds in the presence of light. For example, photo-fermentation with Rhodobacter sphaeroides SH2C can be employed to convert small molecular fatty acids into hydrogen.[190] Electrohydrogenesis is used in microbial fuel cells where hydrogen is produced from organic matter (e.g. from sewage, or solid matter[191]) while 0.2 - 0.8 V is applied.
Biological hydrogen can be produced in an algae bioreactor. In the late 1990s it was discovered that if the algae is deprived of sulfur it will switch from the production of oxygen, i.e. normal photosynthesis, to the production of hydrogen.[192]
Biological hydrogen can be produced in bioreactors that use feedstocks other than algae, the most common feedstock being waste streams. The process involves bacteria feeding on hydrocarbons and excreting hydrogen and CO2. The CO2 can be sequestered successfully by several methods, leaving hydrogen gas. In 2006–2007, NanoLogix first demonstrated a prototype hydrogen bioreactor using waste as a feedstock at Welch's grape juice factory in North East, Pennsylvania (U.S.).[193]
Biocatalysed electrolysis
Besides regular electrolysis, electrolysis using microbes is another possibility. With biocatalysed electrolysis, hydrogen is generated after running through the microbial fuel cell and a variety of aquatic plants can be used. These include reed sweetgrass, cordgrass, rice, tomatoes, lupines, and algae[194]
High-pressure electrolysis
High pressure electrolysis is the electrolysis of water by decomposition of water (H2O) into oxygen (O2) and hydrogen gas (H2) by means of an electric current being passed through the water. The difference with a standard electrolyzer is the compressed hydrogen output around 120-200 bar (1740-2900 psi, 12–20 MPa).[195] By pressurising the hydrogen in the electrolyser, through a process known as chemical compression, the need for an external hydrogen compressor is eliminated,[196] the average energy consumption for internal compression is around 3%.[197] European largest (1 400 000 kg/a, High-pressure Electrolysis of water, alkaline technology) hydrogen production plant is operating at Kokkola, Finland.[198]
High-temperature electrolysis
Hydrogen can be generated from energy supplied in the form of heat and electricity through high-temperature electrolysis (HTE). Because some of the energy in HTE is supplied in the form of heat, less of the energy must be converted twice (from heat to electricity, and then to chemical form), and so potentially far less energy is required per kilogram of hydrogen produced.
While nuclear-generated electricity could be used for electrolysis, nuclear heat can be directly applied to split hydrogen from water. High temperature (950–1000 °C) gas cooled nuclear reactors have the potential to split hydrogen from water by thermochemical means using nuclear heat. Research into high-temperature nuclear reactors may eventually lead to a hydrogen supply that is cost-competitive with natural gas steam reforming. General Atomics predicts that hydrogen produced in a High Temperature Gas Cooled Reactor (HTGR) would cost $1.53/kg. In 2003, steam reforming of natural gas yielded hydrogen at $1.40/kg. In 2005 natural gas prices, hydrogen costs $2.70/kg.
High-temperature electrolysis has been demonstrated in a laboratory, at 108 MJ (thermal) per kilogram of hydrogen produced,[199] but not at a commercial scale. In addition, this is lower-quality "commercial" grade Hydrogen, unsuitable for use in fuel cells.[200]
Photoelectrochemical water splitting
Using electricity produced by photovoltaic systems offers the cleanest way to produce hydrogen. Water is broken into hydrogen and oxygen by electrolysis – a photoelectrochemical cell (PEC) process which is also named artificial photosynthesis.[201] William Ayers at Energy Conversion Devices demonstrated and patented the first multijunction high efficiency photoelectrochemical system for direct splitting of water in 1983.[202] This group demonstrated direct water splitting now referred to as an "artificial leaf" or "wireless solar water splitting" with a low cost thin film amorphous silicon multijunction sheet immersed directly in water.[203][204]
Hydrogen evolved on the front amorphous silicon surface decorated with various catalysts while oxygen evolved off the back metal substrate. A Nafion membrane above the multijunction cell provided a path for ion transport. Their patent also lists a variety of other semiconductor multijunction materials for the direct water splitting in addition to amorphous silicon and silicon germanium alloys. Research continues towards developing high-efficiency multi-junction cell technology at universities and the photovoltaic industry. If this process is assisted by photocatalysts suspended directly in water instead of using photovoltaic and an electrolytic system, the reaction is in just one step, which can improve efficiency.[203][204]
Photoelectrocatalytic production
A method studied by Thomas Nann and his team at the University of East Anglia consists of a gold electrode covered in layers of indium phosphide (InP) nanoparticles. They introduced an iron-sulfur complex into the layered arrangement, which when submerged in water and irradiated with light under a small electric current, produced hydrogen with an efficiency of 60%.[205]
In 2015, it was reported that Panasonic Corp. has developed a photocatalyst based on niobium nitride that can absorb 57% of sunlight to support the decomposition of water to produce hydrogen gas.[206] The company plans to achieve commercial application "as early as possible", not before 2020.
Concentrating solar thermal
Very high temperatures are required to dissociate water into hydrogen and oxygen. A catalyst is required to make the process operate at feasible temperatures. Heating the water can be achieved through the use of water concentrating solar power. Hydrosol-2 is a 100-kilowatt pilot plant at the Plataforma Solar de Almería in Spain which uses sunlight to obtain the required 800 to 1,200 °C to heat water. Hydrosol II has been in operation since 2008. The design of this 100-kilowatt pilot plant is based on a modular concept. As a result, it may be possible that this technology could be readily scaled up to the megawatt range by multiplying the available reactor units and by connecting the plant to heliostat fields (fields of sun-tracking mirrors) of a suitable size.[207]
Thermochemical production
There are more than 352[208] thermochemical cycles which can be used for water splitting,[209] around a dozen of these cycles such as the iron oxide cycle, cerium(IV) oxide-cerium(III) oxide cycle, zinc zinc-oxide cycle, sulfur-iodine cycle, copper-chlorine cycle and hybrid sulfur cycle, aluminum aluminum-oxide cycle, are under research and in testing phase to produce hydrogen and oxygen from water and heat without using electricity.[210] These processes can be more efficient than high-temperature electrolysis, typical in the range from 35% - 49% LHV efficiency. Thermochemical production of hydrogen using chemical energy from coal or natural gas is generally not considered, because the direct chemical path is more efficient.
None of the thermochemical hydrogen production processes have been demonstrated at production levels, although several have been demonstrated in laboratories.
Microwaving plastics
A 97% recovery of hydrogen has been achieved through microwaving plastics for a few seconds that have been ground and mixed with iron oxide and aluminium oxide.[211]
Kværner process
The Kværner process or Kvaerner carbon black and hydrogen process (CB&H)[212] is a method, developed in the 1980s by a Norwegian company of the same name, for the production of hydrogen from hydrocarbons (CnHm), such as methane, natural gas and biogas. Of the available energy of the feed, approximately 48% is contained in the hydrogen, 40% is contained in activated carbon and 10% in superheated steam.[213]
Éric_Claude_Gaucher=== Extraction of naturally-occurring hydrogen === As of 2019, hydrogen is mainly used as an industrial feedstock, primarily for the production of ammonia and methanol, and in petroleum refining. Although initially hydrogen gas was thought not to occur naturally in convenient reservoirs, it is now demonstrated that this is not the case; a hydrogen system is currently being exploited near Bourakebougou, Koulikoro Region in Mali, producing electricity for the surrounding villages.[214] More discoveries of naturally occurring hydrogen in continental, on-shore geological environments have been made in recent years[215] and open the way to the novel field of natural or native hydrogen, supporting energy transition efforts.[216][217]
See also
- Alternative fuel
- Combined cycle hydrogen power plant
- Combined cycle powered railway locomotive
- Energy development
- Fuel Cells and Hydrogen Joint Technology Initiative
- Formic acid
- Hydrogen damage
- Hydrogen embrittlement
- Hydrogen fuel cell power plant
- Hydrogen internal combustion engine vehicle
- Hydrogen prize
- Hydrogen-powered aircraft
- Hydrogen tanker
- International Journal of Hydrogen Energy
- Lolland Hydrogen Community
- Methane pyrolysis
- United States Hydrogen Policy
Notes
- Where data provided in other currencies or units, the average exchange rate to US dollars in the given year are used, and 1kg of H2 is assumed to have a calorific value of 33.3kWh.
References
- International Renewable Energy Agency (2022-03-29). "World Energy Transitions Outlook 1-5C Pathway 2022 edition". IRENA. p. 227. Retrieved 2023-10-06.
- Yap, Jiazhen; McLellan, Benjamin (6 January 2023). "A Historical Analysis of Hydrogen Economy Research, Development, and Expectations, 1972 to 2020". Environments. 10 (1): 11. doi:10.3390/environments10010011. ISSN 2076-3298.
- Greenhouse gas emissions totalled 49.3 Gigatonnes CO2e in 2021."Global Greenhouse Gas Emissions: 1990-2020 and Preliminary 2021 Estimates". Rhodium Group. Retrieved 2023-09-21.
- "Hydrogen". IEA. 10 July 2023. "Energy" section. Retrieved 2023-09-21.
- IEA (2022). Global Hydrogen Review 2022. International Energy Agency. Retrieved 2023-08-25.
- IPCC (2022). Shukla, P.R.; Skea, J.; Slade, R.; Al Khourdajie, A.; et al. (eds.). Climate Change 2022: Mitigation of Climate Change (PDF). Contribution of Working Group III to the Sixth Assessment Report of the Intergovernmental Panel on Climate Change. Cambridge, UK and New York, NY, US: Cambridge University Press (In Press). pp. 91–92. doi:10.1017/9781009157926.
- IPCC (2022). Shukla, P.R.; Skea, J.; Slade, R.; Al Khourdajie, A.; et al. (eds.). Climate Change 2022: Mitigation of Climate Change (PDF). Contribution of Working Group III to the Sixth Assessment Report of the Intergovernmental Panel on Climate Change. Cambridge, UK and New York, NY, US: Cambridge University Press (In Press). pp. 91–92. doi:10.1017/9781009157926.
- IRENA (2021). "World Energy Transitions Outlook: 1.5°C Pathway". International Renewable Energy Agency. Abu Dhabi. p. 95. Retrieved 2023-09-21.
- Plötz, Patrick (2022-01-31). "Hydrogen technology is unlikely to play a major role in sustainable road transport". Nature Electronics. 5 (1): 8–10. doi:10.1038/s41928-021-00706-6. ISSN 2520-1131.
- Rosenow, Jan (September 2022). "Is heating homes with hydrogen all but a pipe dream? An evidence review". Joule. 6 (10): 2225–2228. doi:10.1016/j.joule.2022.08.015.
- Goldman Sachs Research. "Carbonomics: The Clean Hydrogen Revolution". Goldman Sachs. pp. 4–6. Retrieved 2023-09-25.
- "Daedalus or Science and the Future, A paper read to the Heretics, Cambridge, on February 4th, 1923 – Transcript 1993". Archived from the original on 2017-11-15. Retrieved 2016-01-16.
- National Hydrogen Association; United States Department of Energy. "The History of Hydrogen" (PDF). hydrogenassociation.org. National Hydrogen Association. p. 1. Archived from the original (PDF) on 14 July 2010. Retrieved 17 December 2010.
- Jones, Lawrence W (13 March 1970). Toward a liquid hydrogen fuel economy. University of Michigan Environmental Action for Survival Teach In. Ann Arbor, Michigan: University of Michigan. hdl:2027.42/5800.
- Jones, Lawrence W. (March 13, 1970). Toward a Liquid Hydrogen Fuel Economy (PDF). pp. 2–3.
- Bakker, Sjoerd (2010). "The car industry and the blow-out of the hydrogen hype" (PDF). Energy Policy. 38 (11): 6540–6544. doi:10.1016/j.enpol.2010.07.019. Archived (PDF) from the original on 2018-11-03. Retrieved 2019-12-11.
- Harrison, James. "Reactions: Hydrogen hype". Chemical Engineer. 58: 774–775. Archived from the original on 2021-02-08. Retrieved 2017-08-31.
- Rizzi, Francesco Annunziata, Eleonora Liberati, Guglielmo Frey, Marco (2014). "Technological trajectories in the automotive industry: are hydrogen technologies still a possibility?". Journal of Cleaner Production. 66: 328–336. doi:10.1016/j.jclepro.2013.11.069.
{{cite journal}}
: CS1 maint: multiple names: authors list (link) - "Can a viable industry emerge from the hydrogen shakeout?". The Economist. ISSN 0013-0613. Retrieved 2023-09-26.
- Murai, Shusuke (2018-03-05). "Japan's top auto and energy firms tie up to promote development of hydrogen stations". The Japan Times Online. Japan Times. Archived from the original on 2018-04-17. Retrieved 16 April 2018.
- Mishra, Ankit (2018-03-29). "Prospects of fuel-cell electric vehicles boosted with Chinese backing". Energy Post. Archived from the original on 2018-04-17. Retrieved 16 April 2018.
- Plötz, Patrick (January 2022). "Hydrogen technology is unlikely to play a major role in sustainable road transport". Nature Electronics. 5 (1): 8–10. doi:10.1038/s41928-021-00706-6. ISSN 2520-1131.
- Collins (l_collins), Leigh (2022-02-02). "'Hydrogen unlikely to play major role in road transport, even for heavy trucks': Fraunhofer". Recharge. Retrieved 2023-09-08.
- Chu, Yidan; Cui, Hongyang. Annual update on the global transition to electric vehicles: 2022 (PDF). International Council on Clean Transportation. pp. 2–3. Retrieved 2023-08-25.
- Global EV Outlook 2023. IEA. pp. 14–24. Retrieved 2023-08-25.
- IPCC (2022). Shukla, P.R.; Skea, J.; Slade, R.; Al Khourdajie, A.; et al. (eds.). Climate Change 2022: Mitigation of Climate Change (PDF). Contribution of Working Group III to the Sixth Assessment Report of the Intergovernmental Panel on Climate Change. Cambridge, UK and New York, NY, US: Cambridge University Press (In Press). pp. 91–92. doi:10.1017/9781009157926.
- Kjellberg-Motton, Brendan (2022-02-07). "Steel decarbonisation gathers speed | Argus Media". www.argusmedia.com. Retrieved 2023-09-07.
- IPCC (2022). Shukla, P.R.; Skea, J.; Slade, R.; Al Khourdajie, A.; et al. (eds.). Climate Change 2022: Mitigation of Climate Change (PDF). Contribution of Working Group III to the Sixth Assessment Report of the Intergovernmental Panel on Climate Change. Cambridge, UK and New York, NY, US: Cambridge University Press (In Press). pp. 91–92. doi:10.1017/9781009157926.
- Blank, Thomas; Molly, Patrick (January 2020). "Hydrogen's Decarbonization Impact for Industry" (PDF). Rocky Mountain Institute. pp. 2, 7, 8. Archived (PDF) from the original on 22 September 2020.
- IRENA (2021). "World Energy Transitions Outlook: 1.5°C Pathway". International Renewable Energy Agency. Abu Dhabi. p. 95. Retrieved 2023-09-21.
- "Hydrogen Generation Market Size, Share & Trends Analysis Report, 2023 - 2030". www.grandviewresearch.com. Retrieved 2023-08-30.
- "Executive summary – Global Hydrogen Review 2022 – Analysis". IEA. Retrieved 2023-09-21.
- "Hydrogen". IEA. 10 July 2023. "Energy" section. Retrieved 2023-09-21.
- "Hydrogen". IEA. Retrieved 2023-09-21.
- Energy-related emissions totalled 36.3 Gigatonnes CO2 in 2021."Global CO2 emissions rebounded to their highest level in history in 2021 - News". IEA. Retrieved 2023-09-21.
- Greenhouse gas emissions totalled 49.3 Gigatonnes CO2e in 2021."Global Greenhouse Gas Emissions: 1990-2020 and Preliminary 2021 Estimates". Rhodium Group. Retrieved 2023-09-21.
- IEA (2022). Global Hydrogen Review 2022. International Energy Agency. Retrieved 2023-08-25.
- Rosenow, Jan (27 September 2022). "Is heating homes with hydrogen all but a pipe dream? An evidence review". Joule. 6 (10): 2225–2228. doi:10.1016/j.joule.2022.08.015. ISSN 2542-4785. S2CID 252584593. Retrieved 2022-09-29. Article in press.
- national grid. "The hydrogen colour spectrum". National Grid Group. London, United Kingdom. Retrieved 2022-09-29.
- "What potential for natural hydrogen?". Energy Observer. Retrieved 2023-07-03.
- BMWi (June 2020). The national hydrogen strategy (PDF). Berlin, Germany: Federal Ministry for Economic Affairs and Energy (BMWi). Archived (PDF) from the original on 2020-12-13. Retrieved 2020-11-27.
- Van de Graaf, Thijs; Overland, Indra; Scholten, Daniel; Westphal, Kirsten (December 2020). "The new oil? The geopolitics and international governance of hydrogen". Energy Research & Social Science. 70: 101667. doi:10.1016/j.erss.2020.101667. PMC 7326412. PMID 32835007.
- Sansom, Robert; Baxter, Jenifer; Brown, Andy; Hawksworth, Stuart; McCluskey, Ian (2020). Transitioning to hydrogen: assessing the engineering risks and uncertainties (PDF). London, United Kingdom: The Institution of Engineering and Technology (IET). Archived (PDF) from the original on 2020-05-08. Retrieved 2020-03-22.
- Bruce, S; Temminghoff, M; Hayward, J; Schmidt, E; Munnings, C; Palfreyman, D; Hartley, P (2018). National hydrogen roadmap: pathways to an economically sustainable hydrogen industry in Australia (PDF). Australia: CSIRO. Archived (PDF) from the original on 2020-12-08. Retrieved 2020-11-28.
- Department of Earth Sciences (12 September 2022). "Gold hydrogen". Department of Earth Sciences, Oxford University. Oxford, United Kingdom. Retrieved 2022-09-29.
- "Natural Hydrogen Energy LLC". Archived from the original on 2020-10-25. Retrieved 2020-09-29.
- "Definition of Green Hydrogen" (PDF). Clean Energy Partnership. Retrieved 2014-09-06.
- Schneider, Stefan; Bajohr, Siegfried; Graf, Frank; Kolb, Thomas (October 2020). "State of the Art of Hydrogen Production via Pyrolysis of Natural Gas". ChemBioEng Reviews. 7 (5): 150–158. doi:10.1002/cben.202000014.
- Sampson2019-02-11T10:48:00+00:00, Joanna (11 February 2019). "Blue hydrogen for a green future". gasworld. Archived from the original on 2019-05-09. Retrieved 2019-06-03.
- "Brown coal the hydrogen economy stepping stone | ECT". Archived from the original on 2019-04-08. Retrieved 2019-06-03.
- "Actual Worldwide Hydrogen Production from …". Arno A Evers. December 2008. Archived from the original on 2015-02-02. Retrieved 2008-05-09.
- Velazquez Abad, A.; Dodds, P. E. (2017-01-01), Abraham, Martin A. (ed.), "Production of Hydrogen", Encyclopedia of Sustainable Technologies, Oxford: Elsevier, pp. 293–304, ISBN 978-0-12-804792-7, retrieved 2023-09-22
- Badwal, Sukhvinder P. S.; Giddey, Sarbjit S.; Munnings, Christopher; Bhatt, Anand I.; Hollenkamp, Anthony F. (24 September 2014). "Emerging electrochemical energy conversion and storage technologies". Frontiers in Chemistry. 2: 79. Bibcode:2014FrCh....2...79B. doi:10.3389/fchem.2014.00079. PMC 4174133. PMID 25309898.
- Werner Zittel; Reinhold Wurster (1996-07-08). "Chapter 3: Production of Hydrogen. Part 4: Production from electricity by means of electrolysis". HyWeb: Knowledge - Hydrogen in the Energy Sector. Ludwig-Bölkow-Systemtechnik GmbH. Archived from the original on 2007-02-07. Retrieved 2010-10-01.
- Bjørnar Kruse; Sondre Grinna; Cato Buch (2002-02-13). "Hydrogen – Status and Possibilities". The Bellona Foundation. Archived from the original (PDF) on 2011-07-02.
Efficiency factors for PEM electrolysers up to 94% are predicted, but this is only theoretical at this time.
- "high-rate and high efficiency 3D water electrolysis". Grid-shift.com. Archived from the original on 2012-03-22. Retrieved 2011-12-13.
- "Wide Spread Adaption of Competitive Hydrogen Solution" (PDF). nelhydrogen.com. Nel ASA. Archived (PDF) from the original on 2018-04-22. Retrieved 22 April 2018.
- Philibert, Cédric. "Commentary: Producing industrial hydrogen from renewable energy". iea.org. International Energy Agency. Archived from the original on 22 April 2018. Retrieved 22 April 2018.
- IEA H2 2019, p. 37
- "How Much Electricity/Water Is Needed to Produce 1 kg of H2 by Electrolysis?". Archived from the original on 17 June 2020. Retrieved 17 June 2020.
- http://www.nedstack.com/images/stories/news/documents/20120202_Press%20release%20Solvay%20PEM%20Power%20Plant%20start%20up.pdf Archived 2014-12-08 at the Wayback Machine Nedstack
- "Different Gases from Steel Production Processes". Archived from the original on 27 March 2016. Retrieved 5 July 2020.
- "Production of Liquefied Hydrogen Sourced by COG" (PDF). Archived (PDF) from the original on 8 February 2021. Retrieved 8 July 2020.
- Lewis, Alastair C. (10 June 2021). "Optimising air quality co-benefits in a hydrogen economy: a case for hydrogen-specific standards for NO x emissions". Environmental Science: Atmospheres. 1 (5): 201–207. doi:10.1039/D1EA00037C.
This article incorporates text from this source, which is available under the CC BY 3.0 license.
- IPCC (2022). Shukla, P.R.; Skea, J.; Slade, R.; Al Khourdajie, A.; et al. (eds.). Climate Change 2022: Mitigation of Climate Change (PDF). Contribution of Working Group III to the Sixth Assessment Report of the Intergovernmental Panel on Climate Change. Cambridge, UK and New York, NY, US: Cambridge University Press (In Press). p. 1184. doi:10.1017/9781009157926.
- Kjellberg-Motton, Brendan (2022-02-07). "Steel decarbonisation gathers speed | Argus Media". www.argusmedia.com. Retrieved 2023-09-07.
- Blank, Thomas; Molly, Patrick (January 2020). "Hydrogen's Decarbonization Impact for Industry" (PDF). Rocky Mountain Institute. pp. 2, 7, 8. Archived (PDF) from the original on 22 September 2020.
- "This company may have solved one of the hardest problems in clean energy". Vox. 2018-02-16. Archived from the original on 2019-11-12. Retrieved 9 February 2019.
- IRENA. "The Hydrogen Factor". irena.org. Retrieved 2022-10-19.
- "Sustainable fuels and their role in decarbonizing energy | McKinsey". www.mckinsey.com. Retrieved 2022-10-19.
- Spiryagin, Maksym; Dixon, Roger; Oldknow, Kevin; Cole, Colin (2021-09-01). "Preface to special issue on hybrid and hydrogen technologies for railway operations". Railway Engineering Science. 29 (3): 211. doi:10.1007/s40534-021-00254-x. ISSN 2662-4753. S2CID 240522190.
- IPCC (2022). Shukla, P.R.; Skea, J.; Slade, R.; Al Khourdajie, A.; et al. (eds.). Climate Change 2022: Mitigation of Climate Change (PDF). Contribution of Working Group III to the Sixth Assessment Report of the Intergovernmental Panel on Climate Change. Cambridge, UK and New York, NY, US: Cambridge University Press (In Press). pp. 91–92. doi:10.1017/9781009157926.
- Chu, Yidan; Cui, Hongyang. Annual update on the global transition to electric vehicles: 2022 (PDF). International Council on Clean Transportation. pp. 2–3. Retrieved 2023-08-25.
- Global EV Outlook 2023. IEA. pp. 14–24. Retrieved 2023-08-25.
- Plötz, Patrick (January 2022). "Hydrogen technology is unlikely to play a major role in sustainable road transport". Nature Electronics. 5 (1): 8–10. doi:10.1038/s41928-021-00706-6. ISSN 2520-1131.
- Collins (l_collins), Leigh (2022-02-02). "'Hydrogen unlikely to play major role in road transport, even for heavy trucks': Fraunhofer". Recharge. Retrieved 2023-09-08.
- World energy outlook 2022. International Energy Agency. p. 150.
This article incorporates text from this source, which is available under the CC BY 4.0 license.
- Cozzi, Laura; Gould, Tim. World Energy Outlook 2022 (PDF). International Energy Agency. pp. Page 148.
This article incorporates text from this source, which is available under the CC BY 4.0 license.
- Collins, Leigh (2021-12-10). "Even the European gas lobby can't make a case for hydrogen boilers — so why does it say gases are needed to decarbonise heating?". Recharge | Latest renewable energy news. Retrieved 2023-09-25.
- Roth, Sammy (2023-02-09). "California declared war on natural gas. Now the fight is going national". Los Angeles Times. Retrieved 2023-09-25.
- Rosenow, Jan (September 2022). "Is heating homes with hydrogen all but a pipe dream? An evidence review". Joule. 6 (10): 2225–2228. doi:10.1016/j.joule.2022.08.015.
- Schrotenboer, Albert H.; Veenstra, Arjen A.T.; uit het Broek, Michiel A.J.; Ursavas, Evrim (October 2022). "A Green Hydrogen Energy System: Optimal control strategies for integrated hydrogen storage and power generation with wind energy" (PDF). Renewable and Sustainable Energy Reviews. 168: 112744. doi:10.1016/j.rser.2022.112744. S2CID 250941369.
- Lipták, Béla (January 24, 2022). "Hydrogen is key to sustainable green energy". Control. Retrieved February 12, 2023.
- Agosta, Vito (July 10, 2003). "The Ammonia Economy". Archived from the original on May 13, 2008. Retrieved 2008-05-09.
- "Renewable Energy". Iowa Energy Center. Archived from the original on 2008-05-13. Retrieved 2008-05-09.
- UKCCC H2 2018, p. 79: The potential for bio-gasification with CCS to be deployed at scale is limited by the amount of sustainable bioenergy available. .... "
- UKCCC H2 2018, p. 33: production of biofuels, even with CCS, is only one of the best uses of the finite sustainable bio-resource if the fossil fuels it displaces cannot otherwise feasibly be displaced (e.g. use of biomass to produce aviation biofuels with CCS)."
- Bjørnæs, Christian. "Global warming potential of hydrogen estimated", Centre for International Climate and Environmental Research, June 7, 2023. Retrieved June 15, 2023
- Zubrin, Robert (2007). Energy Victory. Amherst, New York: Prometheus Books. pp. 117–118. ISBN 978-1-59102-591-7.
The situation is much worse than this, however, because before the hydrogen can be transported anywhere, it needs to be either compressed or liquefied. To liquefy it, it must be refrigerated down to a temperature of -253°C (20 degrees above absolute zero). At these temperatures, fundamental laws of thermodynamics make refrigerators extremely inefficient. As a result, about 40 percent of the energy in the hydrogen must be spent to liquefy it. This reduces the actual net energy content of our product fuel to 792 kcal. In addition, because it is a cryogenic liquid, still more energy could be expected to be lost as the hydrogen boils away as it is warmed by heat leaking in from the outside environment during transport and storage.
- Savvides, Nick (2017-01-11). "Japan plans to use imported liquefied hydrogen to fuel Tokyo 2020 Olympics". Safety At Sea. IHS Markit Maritime Portal. Archived from the original on 2018-04-23. Retrieved 22 April 2018.
- S.Sadaghiani, Mirhadi (2 March 2017). "Introducing and energy analysis of a novel cryogenic hydrogen liquefaction process configuration". International Journal of Hydrogen Energy. 42 (9).
- 1994 – ECN abstract Archived 2004-01-02 at the Wayback Machine. Hyweb.de. Retrieved on 2012-01-08.
- European Renewable Energy Network Archived 2019-07-17 at the Wayback Machine pp. 86, 188
- "Energy storage – the role of electricity" (PDF). European Commission. Archived from the original (PDF) on 8 November 2020. Retrieved 22 April 2018.
- "Hyunder". Archived from the original on 2013-11-11. Retrieved 2013-11-11.
- "Storing renewable energy: Is hydrogen a viable solution?" (PDF).
- "BRINGING NORTH SEA ENERGY ASHORE EFFICIENTLY" (PDF). worldenergy.org. World Energy Council Netherlands. Archived (PDF) from the original on 23 April 2018. Retrieved 22 April 2018.
- GERDES, JUSTIN (2018-04-10). "Enlisting Abandoned Oil and Gas Wells as 'Electron Reserves'". greentechmedia.com. Wood MacKenzie. Archived from the original on 2018-04-23. Retrieved 22 April 2018.
- Bhadhesia, Harry. "Prevention of Hydrogen Embrittlement in Steels" (PDF). Phase Transformations & Complex Properties Research Group, Cambridge University. Archived (PDF) from the original on 11 November 2020. Retrieved 17 December 2020.
- IEA H2 2019, p. 15
- "Japan's Hydrogen Strategy and Its Economic and Geopolitical Implications". Etudes de l'Ifri. Archived from the original on 10 February 2019. Retrieved 9 February 2019.
- "South Korea's Hydrogen Economy Ambitions". The Diplomat. Archived from the original on 9 February 2019. Retrieved 9 February 2019.
- "The world's largest-class hydrogen production, Fukushima Hydrogen Energy Research Field (FH2R) now is completed at Namie town in Fukushima". Toshiba Energy Press Releases. Toshiba Energy Systems and Solutions Corporations. 7 March 2020. Archived from the original on 22 April 2020. Retrieved 1 April 2020.
- Patel, Sonal (2022-07-01). "Fukushima Hydrogen Energy Research Field Demonstrates Hydrogen Integration". POWER Magazine. Retrieved 2023-10-05.
- Utgikar, Vivek P; Thiesen, Todd (2005). "Safety of compressed hydrogen fuel tanks: Leakage from stationary vehicles". Technology in Society. 27 (3): 315–320. doi:10.1016/j.techsoc.2005.04.005.
- Cadwallader, L. C.; Herring, J. S. (1999). Safety Issues with Hydrogen as a Vehicle Fuel (Technical report). doi:10.2172/761801.
- "AIAA G-095-2004, Guide to Safety of Hydrogen and Hydrogen Systems" (PDF). AIAA. Retrieved 2008-07-28.
- "List of NFPA Codes & Standards". NFPA.
- "Standards and/or projects under the direct responsibility of ISO/TC 197 Secretariat of the International Organization for Standardization".
- "Canadian Hydrogen Safety Program testing H2/CNG". Hydrogenandfuelcellsafety.info. Archived from the original on 2011-07-21. Retrieved 2010-07-05.
- Making the Hydrogen Economy Possible: Accelerating Clean Hydrogen in an Electrified Economy. Energy Transitions Commission. April 2021. Retrieved 2023-08-25.
- Global Hydrogen Review 2022. IEA. p. 93. Retrieved 2023-08-25.
- PricewaterhouseCoopers. "Green hydrogen economy - predicted development of tomorrow". PwC. Retrieved 2023-08-25.
- "Hydrogen Production Costs 2021 annex: Key assumptions and outputs for production technologies". GOV.UK. Retrieved 2023-08-25.
- Saini, Anshuman (January 12, 2023). "Green & Blue Hydrogen: Current Levelized Cost of Production & Outlook | GEP Blogs". www.gep.com. Retrieved 2023-08-25.
- IRENA (2020), Green Hydrogen Cost Reduction: Scaling up Electrolysers to Meet the 1.5⁰C Climate Goal, International Renewable Energy Agency, Abu Dhabi, p. 91.
- 2023 Levelized Cost Of Energy+. Lazard. April 12, 2023. p. 27. Retrieved 2023-08-25.
- Patonia, Aliaksei; Poudineh, Rahmat (January 2022). Cost-competitive green hydrogen: how to lower the cost of electrolysers?. Oxford Institute for Energy Studies. Retrieved 2023-08-25.
- Roser, Max (2023-09-01). "Why did renewables become so cheap so fast?". Our World in Data.
- Goldman Sachs Research. "Carbonomics: The Clean Hydrogen Revolution". Goldman Sachs. pp. 4–6. Retrieved 2023-09-25.
- Martin, Polly (2023-06-29). "India to offer green hydrogen production subsidy of up to $0.60/kg — for three years only". Hydrogen news and intelligence | Hydrogen Insight. Retrieved 2023-09-26.
- "Are hydrogen fuel cell vehicles the future of autos?". ABC News. Archived from the original on 2021-01-17. Retrieved 2021-01-18.
- Siddiqui, Faiz. "The plug-in electric car is having its moment. But despite false starts, Toyota is still trying to make the fuel cell happen". Washington Post. ISSN 0190-8286. Archived from the original on 2021-01-19. Retrieved 2021-01-18.
- "Experimental 'wind to hydrogen' system up and running". Physorg.com. January 8, 2007. Archived from the original on 2013-01-26. Retrieved 2008-05-09.
- "Hydrogen Engine Center Receives Order for Hydrogen Power Generator 250kW Generator for Wind/Hydrogen Demonstration" (PDF). Hydrogen Engine Center, Inc. May 16, 2006. Archived from the original (PDF) on May 27, 2008. Retrieved 2008-05-09.
- "Stuart Island Energy Initiative". Archived from the original on 2013-06-18. Retrieved 2008-05-09.
- "Hydrogen transport & distribution". Archived from the original on 2019-09-29. Retrieved 2019-09-29.
- Pollet, Mathieu (2020). "AExplainer: Why is the EU Commission betting on hydrogen for a greener future?". euronews. Archived from the original on 2020-08-07. Retrieved 2020-08-14.
- "ECHA". Archived from the original on 2020-08-12. Retrieved 2020-08-14.
- "Hydrogen buses". Transport for London. Archived from the original on March 23, 2008. Retrieved 2008-05-09.
- "The Hydrogen Expedition" (PDF). January 2005. Archived from the original (PDF) on 2008-05-27. Retrieved 2008-05-09.
- "UK Hydrogen Strategy" (PDF). UK Government. August 2021. Archived (PDF) from the original on 2021-08-19. Retrieved 2021-08-19.
- "Perth Fuel Cell Bus Trial". Department for Planning and Infrastructure, Government of Western Australia. 13 April 2007. Archived from the original on 7 June 2008. Retrieved 2008-05-09.
- Hannesson, Hjálmar W. (2007-08-02). "Climate change as a global challenge". Iceland Ministry for Foreign Affairs. Archived from the original on 2014-01-07. Retrieved 2008-05-09.
- Doyle, Alister (January 14, 2005). "Iceland's hydrogen buses zip toward oil-free economy". Reuters. Archived from the original on July 24, 2012. Retrieved 2008-05-09.
- "What is HyFLEET:CUTE?". Archived from the original on 2008-02-24. Retrieved 2008-05-09.
- "Hydrogen vehicles and refueling infrastructure in India" (PDF). Archived (PDF) from the original on 2017-06-12. Retrieved 2019-09-28.
- Das, L (1991). "Exhaust emission characterization of hydrogen-operated engine system: Nature of pollutants and their control techniques". International Journal of Hydrogen Energy. 16 (11): 765–775. doi:10.1016/0360-3199(91)90075-T.
- "UK-India Energy Collaborations report" (PDF).
- "MNRE: FAQ". Archived from the original on 2019-09-21. Retrieved 2019-09-28.
- Overview of Indian Hydrogen Programme
- "H2 stations worldwide". Archived from the original on 2019-09-21. Retrieved 2019-09-28.
- "India working on more H2 stations". 23 February 2016. Archived from the original on 2019-09-21. Retrieved 2019-09-28.
- "Shell plans to open 1200 fuel stations in India, some of which may include H2 refilling". The Economic Times. Archived from the original on 2019-09-22. Retrieved 2019-09-28.
- "Hydrogen Vehicles and Refueling Infrastructure in India" (PDF). Archived (PDF) from the original on 2017-06-12. Retrieved 2019-09-28.
- "Independent Mid-Term Review of the UNIDO Project: Establishment and operation of the International Centre for Hydrogen Energy Technologies (ICHET), TF/INT/03/002" (PDF). UNIDO. 31 August 2009. Archived from the original (PDF) on 1 June 2010. Retrieved 20 July 2010.
- "Worldwide NGV statistics". Archived from the original on 2015-02-06. Retrieved 2019-09-29.
- "Fuel Cell micro CHP". Archived from the original on 2019-11-06. Retrieved 2019-10-23.
- "Fuel cell micro Cogeneration". Archived from the original on 2019-10-23. Retrieved 2019-10-23.
- "'Green industrial revolution': Queensland announces plans to mass produce green ammonia". ABC News. October 11, 2021. Archived from the original on 2021-10-12. Retrieved 2021-10-12 – via www.abc.net.au.
- "E3B1C256-BFCB-4CEF-88A6-1DCCD7666635". 24 October 2007. Archived from the original on 2021-10-29. Retrieved 2021-10-12.
- "Saudi Arabia's $5bn green hydrogen-based ammonia plant to begin production in 2025". Energy & Utilities. 21 April 2021. Retrieved 2022-01-13.
- "Australia's pathway to $2 per kg hydrogen - ARENAWIRE". Australian Renewable Energy Agency. 30 November 2020. Archived from the original on 2020-12-15. Retrieved 2021-01-06.
- Ambrose, Jillian (20 August 2021). "Oil firms made 'false claims' on blue hydrogen costs, says ex-lobby boss". The Guardian. London, United Kingdom. ISSN 0261-3077. Archived from the original on 2021-08-24. Retrieved 2021-08-24.
- "French port bets big on floating wind farms planned in Mediterranean". European Investment Bank. Retrieved 2022-09-26.
- "Green Hydrogen: A key investment for the energy transition". blogs.worldbank.org. 23 June 2022. Retrieved 2022-09-26.
- "Australian researchers claim 'giant leap' in technology to produce affordable renewable hydrogen". The Guardian. 16 March 2022. Archived from the original on 28 April 2022. Retrieved 28 April 2022.
- Hodges, Aaron; Hoang, Anh Linh; Tsekouras, George; Wagner, Klaudia; Lee, Chong-Yong; Swiegers, Gerhard F.; Wallace, Gordon G. (15 March 2022). "A high-performance capillary-fed electrolysis cell promises more cost-competitive renewable hydrogen". Nature Communications. 13 (1): 1304. Bibcode:2022NatCo..13.1304H. doi:10.1038/s41467-022-28953-x. ISSN 2041-1723. PMC 8924184. PMID 35292657. S2CID 247475206.
- Shipman, Matt. "Driving down the costs of hydrogen fuel: Prototype achieves 99% yield 8 times faster than conventional batch reactors". North Carolina State University. Archived from the original on 8 August 2022. Retrieved 8 August 2022.
- Ibrahim, Malek Y. S.; Bennett, Jeffrey A.; Abolhasani, Milad (21 July 2022). "Continuous Room‐Temperature Hydrogen Release from Liquid Organic Carriers in a Photocatalytic Packed‐Bed Flow Reactor". ChemSusChem. 15 (14): e202200733. doi:10.1002/cssc.202200733. ISSN 1864-5631. PMC 9400973. PMID 35446510.
- "Mechanochemical breakthrough unlocks cheap, safe, powdered hydrogen". New Atlas. 19 July 2022. Archived from the original on 16 August 2022. Retrieved 22 August 2022.
- Mateti, Srikanth; Zhang, Chunmei; Du, Aijun; Periasamy, Selvakannan; Chen, Ying Ian (1 July 2022). "Superb storage and energy saving separation of hydrocarbon gases in boron nitride nanosheets via a mechanochemical process". Materials Today. 57: 26–34. doi:10.1016/j.mattod.2022.06.004. ISSN 1369-7021. S2CID 250413503. Archived from the original on 24 August 2022. Retrieved 30 August 2022.
- Yirka, Bob. "Making hydrogen out of thin air". techxplore.com. Archived from the original on 26 October 2022. Retrieved 26 October 2022.
- Guo, Jining; Zhang, Yuecheng; Zavabeti, Ali; Chen, Kaifei; Guo, Yalou; Hu, Guoping; Fan, Xiaolei; Li, Gang Kevin (6 September 2022). "Hydrogen production from the air". Nature Communications. 13 (1): 5046. Bibcode:2022NatCo..13.5046G. doi:10.1038/s41467-022-32652-y. ISSN 2041-1723. PMC 9448774. PMID 36068193.
- Paleja, Ameya (19 October 2022). "German researchers find a solution to the hydrogen storage problem: salts". interestingengineering.com. Archived from the original on 17 November 2022. Retrieved 17 November 2022.
- Wei, Duo; Shi, Xinzhe; Sponholz, Peter; Junge, Henrik; Beller, Matthias (26 October 2022). "Manganese Promoted (Bi)carbonate Hydrogenation and Formate Dehydrogenation: Toward a Circular Carbon and Hydrogen Economy". ACS Central Science. 8 (10): 1457–1463. doi:10.1021/acscentsci.2c00723. ISSN 2374-7943. PMC 9615124. PMID 36313168.
- Timmer, John (30 November 2022). "New device can make hydrogen when dunked in salt water". Ars Technica. Archived from the original on 18 December 2022. Retrieved 18 December 2022.
- Xie, Heping; Zhao, Zhiyu; Liu, Tao; Wu, Yifan; Lan, Cheng; Jiang, Wenchuan; Zhu, Liangyu; Wang, Yunpeng; Yang, Dongsheng; Shao, Zongping (30 November 2022). "A membrane-based seawater electrolyser for hydrogen generation". Nature. 612 (7941): 673–678. Bibcode:2022Natur.612..673X. doi:10.1038/s41586-022-05379-5. ISSN 1476-4687. PMID 36450987. S2CID 254123372.
- Theresa, Deena (14 December 2022). "Engineers use sound waves to boost green hydrogen production by 14 times". Interesting Engineering. Archived from the original on 2 February 2023. Retrieved 18 January 2023.
- Ehrnst, Yemima; Sherrell, Peter C.; Rezk, Amgad R.; Yeo, Leslie Y. (4 December 2022). "Acoustically‐Induced Water Frustration for Enhanced Hydrogen Evolution Reaction in Neutral Electrolytes". Advanced Energy Materials. 13 (7): 2203164. doi:10.1002/aenm.202203164. ISSN 1614-6832. S2CID 254299691.
- "Sun-powered water splitter produces unprecedented levels of green energy". Science. Archived from the original on 16 February 2023. Retrieved 16 February 2023.
- Yirka, Bob. "A way to produce hydrogen directly from untreated sea water". techxplore.com. Archived from the original on 16 February 2023. Retrieved 16 February 2023.
- Zhou, Peng; Navid, Ishtiaque Ahmed; Ma, Yongjin; Xiao, Yixin; Wang, Ping; Ye, Zhengwei; Zhou, Baowen; Sun, Kai; Mi, Zetian (January 2023). "Solar-to-hydrogen efficiency of more than 9% in photocatalytic water splitting". Nature. 613 (7942): 66–70. Bibcode:2023Natur.613...66Z. doi:10.1038/s41586-022-05399-1. ISSN 1476-4687. PMID 36600066. S2CID 255474993. Archived from the original on 3 February 2023. Retrieved 16 February 2023.
- Guo, Jiaxin; Zheng, Yao; Hu, Zhenpeng; Zheng, Caiyan; Mao, Jing; Du, Kun; Jaroniec, Mietek; Qiao, Shi-Zhang; Ling, Tao (30 January 2023). "Direct seawater electrolysis by adjusting the local reaction environment of a catalyst". Nature Energy: 1–9. doi:10.1038/s41560-023-01195-x. ISSN 2058-7546. S2CID 256493839.
- Young, Chris (14 February 2023). "A new method converts seawater straight into green hydrogen". interestingengineering.com. Archived from the original on 3 April 2023. Retrieved 4 April 2023.
- Loomba, Suraj; Khan, Muhammad Waqas; Haris, Muhammad; Mousavi, Seyed Mahdi; Zavabeti, Ali; Xu, Kai; Tadich, Anton; Thomsen, Lars; McConville, Christopher F.; Li, Yongxiang; Walia, Sumeet; Mahmood, Nasir (8 February 2023). "Nitrogen‐Doped Porous Nickel Molybdenum Phosphide Sheets for Efficient Seawater Splitting". Small. 19 (18): 2207310. doi:10.1002/smll.202207310. PMID 36751959. S2CID 256663170.
- "Gasleitungen in Deutschland sind bereit für Wasserstoff". www.forschung-und-wissen.de (in German). Retrieved 20 April 2023.
- "DVGW: Germany's gas pipelines h2ready". DVGW. Archived from the original on 20 April 2023. Retrieved 20 April 2023.
- "Concentrated solar reactor generates unprecedented amounts of hydrogen". Physics World. 18 May 2023. Archived from the original on 28 May 2023. Retrieved 28 May 2023.
- Holmes-Gentle, Isaac; Tembhurne, Saurabh; Suter, Clemens; Haussener, Sophia (10 April 2023). "Kilowatt-scale solar hydrogen production system using a concentrated integrated photoelectrochemical device". Nature Energy. 8 (6): 586–596. doi:10.1038/s41560-023-01247-2. ISSN 2058-7546.
- Fehr, Austin M. K.; Agrawal, Ayush; Mandani, Faiz; Conrad, Christian L.; Jiang, Qi; Park, So Yeon; Alley, Olivia; Li, Bor; Sidhik, Siraj; Metcalf, Isaac; Botello, Christopher; Young, James L.; Even, Jacky; Blancon, Jean Christophe; Deutsch, Todd G.; Zhu, Kai; Albrecht, Steve; Toma, Francesca M.; Wong, Michael; Mohite, Aditya D. (26 June 2023). "Integrated halide perovskite photoelectrochemical cells with solar-driven water-splitting efficiency of 20.8%". Nature Communications. 14 (1): 3797. Bibcode:2023NatCo..14.3797F. doi:10.1038/s41467-023-39290-y. ISSN 2041-1723. PMC 10293190. PMID 37365175.
- University report: Clark, Silvia Cernea. "Device makes hydrogen from sunlight with record efficiency". techxplore.com. Retrieved 6 September 2023.
- Fernandez, Sonia. "Researchers develop potentially low-cost, low-emissions technology that can convert methane without forming CO2". Phys-Org. American Institute of Physics. Archived from the original on 19 October 2020. Retrieved 19 October 2020.
- BASF. "BASF researchers working on fundamentally new, low-carbon production processes, Methane Pyrolysis". United States Sustainability. BASF. Archived from the original on 19 October 2020. Retrieved 19 October 2020.
- Upham, D. Chester; Agarwal, Vishal; Khechfe, Alexander; Snodgrass, Zachary R.; Gordon, Michael J.; Metiu, Horia; McFarland, Eric W. (17 November 2017). "Catalytic molten metals for the direct conversion of methane to hydrogen and separable carbon". Science. 358 (6365): 917–921. Bibcode:2017Sci...358..917U. doi:10.1126/science.aao5023. PMID 29146810. S2CID 206663568.
- Palmer, Clarke; Upham, D. Chester; Smart, Simon; Gordon, Michael J.; Metiu, Horia; McFarland, Eric W. (January 2020). "Dry reforming of methane catalysed by molten metal alloys". Nature Catalysis. 3 (1): 83–89. doi:10.1038/s41929-019-0416-2. S2CID 210862772.
- Cartwright, Jon. "The reaction that would give us clean fossil fuels forever". NewScientist. New Scientist Ltd. Archived from the original on 26 October 2020. Retrieved 30 October 2020.
- Karlsruhe Institute of Technology. "Hydrogen from methane without CO2 emissions". Phys.Org. Archived from the original on 21 October 2020. Retrieved 30 October 2020.
- Proceedings hcei.tsc.ru
- Lumbers, Brock (2022). "Mathematical modelling and simulation of the thermo-catalytic decomposition of methane for economically improved hydrogen production". International Journal of Hydrogen Energy. 47 (7): 4265–4283. doi:10.1016/j.ijhydene.2021.11.057. S2CID 244814932. Retrieved 16 March 2022.
- Tao, Yongzhen; Chen, Yang; Wu, Yongqiang; He, Yanling; Zhou, Zhihua (1 February 2007). "High hydrogen yield from a two-step process of dark- and photo-fermentation of sucrose". International Journal of Hydrogen Energy. 32 (2): 200–206. doi:10.1016/j.ijhydene.2006.06.034. INIST:18477081.
- "Hydrogen production from organic solid matter". Biohydrogen.nl. Archived from the original on 2011-07-20. Retrieved 2010-07-05.
- Hemschemeier, Anja; Melis, Anastasios; Happe, Thomas (December 2009). "Analytical approaches to photobiological hydrogen production in unicellular green algae". Photosynthesis Research. 102 (2–3): 523–540. doi:10.1007/s11120-009-9415-5. PMC 2777220. PMID 19291418.
- "NanoLogix generates energy on-site with bioreactor-produced hydrogen". Solid State Technology. September 20, 2007. Archived from the original on 2018-05-15. Retrieved 14 May 2018.
- "Power from plants using microbial fuel cell" (in Dutch). Archived from the original on 2021-02-08. Retrieved 2010-07-05.
- Janssen, H; Emonts, B.; Groehn, H. G.; Mai, H.; Reichel, R.; Stolten, D. (1 July 2001). High pressure electrolysis : the key technology for efficient H2 production (PDF). Hypothesis IV: Hydrogen power - theoretical and engineering solutions international symposium.
- Carmo, M; Fritz D; Mergel J; Stolten D (2013). "A comprehensive review on PEM water electrolysis". Journal of Hydrogen Energy. 38 (12): 4901–4934. doi:10.1016/j.ijhydene.2013.01.151.
- "2003-PHOEBUS-Pag.9" (PDF). Archived from the original (PDF) on 2009-03-27. Retrieved 2010-07-05.
- "Finland exporting TEN-T fuel stations". December 2015. Archived from the original on 2016-08-28. Retrieved 2016-08-22.
- "Steam heat: researchers gear up for full-scale hydrogen plant" (Press release). Science Daily. 2008-09-18. Archived from the original on 2008-09-21. Retrieved 2008-09-19.
- "Nuclear Hydrogen R&D Plan" (PDF). U.S. Dept. of Energy. March 2004. Archived from the original (PDF) on 2008-05-18. Retrieved 2008-05-09.
- Valenti, Giovanni; Boni, Alessandro; Melchionna, Michele; Cargnello, Matteo; Nasi, Lucia; Bertoni, Giovanni; Gorte, Raymond J.; Marcaccio, Massimo; Rapino, Stefania; Bonchio, Marcella; Fornasiero, Paolo; Prato, Maurizio; Paolucci, Francesco (December 2016). "Co-axial heterostructures integrating palladium/titanium dioxide with carbon nanotubes for efficient electrocatalytic hydrogen evolution". Nature Communications. 7 (1): 13549. Bibcode:2016NatCo...713549V. doi:10.1038/ncomms13549. PMC 5159813. PMID 27941752.
- William Ayers, US Patent 4,466,869 Photolytic Production of Hydrogen
- Navarro Yerga, Rufino M.; Álvarez Galván, M. Consuelo; del Valle, F.; Villoria de la Mano, José A.; Fierro, José L. G. (22 June 2009). "Water Splitting on Semiconductor Catalysts under Visible-Light Irradiation". ChemSusChem. 2 (6): 471–485. doi:10.1002/cssc.200900018. PMID 19536754.
- Navarro, R.M.; Del Valle, F.; Villoria de la Mano, J.A.; Álvarez-Galván, M.C.; Fierro, J.L.G. (2009). "Photocatalytic Water Splitting Under Visible Light". Advances in Chemical Engineering - Photocatalytic Technologies. Vol. 36. pp. 111–143. doi:10.1016/S0065-2377(09)00404-9. ISBN 978-0-12-374763-1.
- Nann, Thomas; Ibrahim, Saad K.; Woi, Pei-Meng; Xu, Shu; Ziegler, Jan; Pickett, Christopher J. (22 February 2010). "Water Splitting by Visible Light: A Nanophotocathode for Hydrogen Production". Angewandte Chemie International Edition. 49 (9): 1574–1577. doi:10.1002/anie.200906262. PMID 20140925.
- Yamamura, Tetsushi (August 2, 2015). "Panasonic moves closer to home energy self-sufficiency with fuel cells". Asahi Shimbun. Archived from the original on August 7, 2015. Retrieved 2015-08-02.
- "DLR Portal - DLR scientists achieve solar hydrogen production in a 100-kilowatt pilot plant". Dlr.de. 2008-11-25. Archived from the original on 2013-06-22. Retrieved 2009-09-19.
- "353 Thermochemical cycles" (PDF). Archived (PDF) from the original on 2009-02-05. Retrieved 2010-07-05.
- UNLV Thermochemical cycle automated scoring database (public)
- "Development of Solar-powered Thermochemical Production of Hydrogen from Water" (PDF). Archived (PDF) from the original on 2007-04-17. Retrieved 2010-07-05.
- Jie, Xiangyu; Li, Weisong; Slocombe, Daniel; Gao, Yige; Banerjee, Ira; Gonzalez-Cortes, Sergio; Yao, Benzhen; AlMegren, Hamid; Alshihri, Saeed; Dilworth, Jonathan; Thomas, John; Xiao, Tiancun; Edwards, Peter (2020). "Microwave-initiated catalytic deconstruction of plastic waste into hydrogen and high-value carbons" (PDF). Nature Catalysis. 3 (11): 902–912. doi:10.1038/s41929-020-00518-5. ISSN 2520-1158. S2CID 222299492. Archived (PDF) from the original on 2021-07-16. Retrieved 2021-10-24.
- "Bellona-HydrogenReport". Interstatetraveler.us. Archived from the original on 2016-06-03. Retrieved 2010-07-05.
- https://www.hfpeurope.org/infotools/energyinfos__e/hydrogen/main03.html%5B%5D
- Prinzhofer, Alain; Tahara Cissé, Cheick Sidy; Diallo, Aliou Boubacar (October 2018). "Discovery of a large accumulation of natural hydrogen in Bourakebougou (Mali)". International Journal of Hydrogen Energy. 43 (42): 19315–19326. doi:10.1016/j.ijhydene.2018.08.193. S2CID 105839304.
- Larin, Nikolay; Zgonnik, Viacheslav; Rodina, Svetlana; Deville, Eric; Prinzhofer, Alain; Larin, Vladimir N. (September 2015). "Natural Molecular Hydrogen Seepage Associated with Surficial, Rounded Depressions on the European Craton in Russia". Natural Resources Research. 24 (3): 369–383. doi:10.1007/s11053-014-9257-5. S2CID 128762620.
- Gaucher, Eric C. (1 February 2020). "New Perspectives in the Industrial Exploration for Native Hydrogen". Elements. 16 (1): 8–9. doi:10.2138/gselements.16.1.8.
- Truche, Laurent; Bazarkina, Elena F. (2019). "Natural hydrogen the fuel of the 21 st century". E3S Web of Conferences. 98: 03006. Bibcode:2019E3SWC..9803006T. doi:10.1051/e3sconf/20199803006.
Sources
- Hydrogen in a low-carbon economy (PDF). UK Committee on Climate Change. 2018.
- The Future of Hydrogen. International Energy Agency. 2019.
- Hydrogen. International Energy Agency. 2022
External links
Media related to Hydrogen economy at Wikimedia Commons
Quotations related to Hydrogen economy at Wikiquote
- International Partnership for the Hydrogen Economy
- European Hydrogen Association
- Hydrogen energy projects in Australia
- Online calculator for green hydrogen production and transport costs