Chaperone (protein)
In molecular biology, molecular chaperones are proteins that assist the conformational folding or unfolding of large proteins or macromolecular protein complexes. There are a number of classes of molecular chaperones, all of which function to assist large proteins in proper protein folding during or after synthesis, and after partial denaturation. Chaperones are also involved in the translocation of proteins for proteolysis.
The first molecular chaperones discovered were a type of assembly chaperones which assist in the assembly of nucleosomes from folded histones and DNA.[1][2] One major function of molecular chaperones is to prevent the aggregation of misfolded proteins, thus many chaperone proteins are classified as heat shock proteins, as the tendency for protein aggregation is increased by heat stress.
The majority of molecular chaperones do not convey any steric information for protein folding, and instead assist in protein folding by binding to and stabilizing folding intermediates until the polypeptide chain is fully translated. The specific mode of function of chaperones differs based on their target proteins and location. Various approaches have been applied to study the structure, dynamics and functioning of chaperones. Bulk biochemical measurements have informed us on the protein folding efficiency, and prevention of aggregation when chaperones are present during protein folding. Recent advances in single-molecule analysis[3] have brought insights into structural heterogeneity of chaperones, folding intermediates and affinity of chaperones for unstructured and structured protein chains.
Functions of molecular chaperones
Many chaperones are heat shock proteins, that is, proteins expressed in response to elevated temperatures or other cellular stresses.[4] Heat shock protein chaperones are classified based on their observed molecular weights into Hsp60, Hsp70, Hsp90, Hsp104, and small Hsps.[5] The Hsp60 family of protein chaperones are termed chaperonins, and are characterized by a stacked double-ring structure and are found in prokaryotes, in the cytosol of eukaryotes, and in mitochondria.
Some chaperone systems work as foldases: they support the folding of proteins in an ATP-dependent manner (for example, the GroEL/GroES or the DnaK/DnaJ/GrpE system). Although most newly synthesized proteins can fold in absence of chaperones, a minority strictly requires them for the same. Other chaperones work as holdases: they bind folding intermediates to prevent their aggregation, for example DnaJ or Hsp33.[6] Chaperones can also work as disaggregases, which interact with aberrant protein assemblies and revert them to monomers.[7] Some chaperones can assist in protein degradation, leading proteins to protease systems, such as the ubiquitin-proteasome system in eukaryotes.[8] Chaperone proteins participate in the folding of over half of all mammalian proteins.
Macromolecular crowding may be important in chaperone function. The crowded environment of the cytosol can accelerate the folding process, since a compact folded protein will occupy less volume than an unfolded protein chain.[9] However, crowding can reduce the yield of correctly folded protein by increasing protein aggregation.[10][11] Crowding may also increase the effectiveness of the chaperone proteins such as GroEL,[12] which could counteract this reduction in folding efficiency.[13] Some highly specific 'steric chaperones' convey unique structural information onto proteins, which cannot be folded spontaneously. Such proteins violate Anfinsen's dogma,[14] requiring protein dynamics to fold correctly.
Other types of chaperones are involved in transport across membranes, for example membranes of the mitochondria and endoplasmic reticulum (ER) in eukaryotes. A bacterial translocation-specific chaperone SecB maintains newly synthesized precursor polypeptide chains in a translocation-competent (generally unfolded) state and guides them to the translocon.[15]
New functions for chaperones continue to be discovered, such as bacterial adhesin activity, induction of aggregation towards non-amyloid aggregates,[16] suppression of toxic protein oligomers via their clustering,[17][18] and in responding to diseases linked to protein aggregation[19] and cancer maintenance.[20]
Human chaperone proteins
In human cell lines, chaperone proteins were found to compose ~10% of the gross proteome mass,[21] and are ubiquitously and highly expressed across human tissues.
Chaperones are found extensively in the endoplasmic reticulum (ER), since protein synthesis often occurs in this area.
Endoplasmic reticulum
In the endoplasmic reticulum (ER) there are general, lectin- and non-classical molecular chaperones that moderate protein folding.
- General chaperones: GRP78/BiP, GRP94, GRP170.
- Lectin chaperones: calnexin and calreticulin
- Non-classical molecular chaperones: HSP47 and ERp29
- Folding chaperones:
- Protein disulfide isomerase (PDI),[22]
- Peptidyl prolyl cis-trans isomerase (PPI), Prolyl isomerase[23]
- ERp57[24]
Nomenclature and examples of chaperone families
There are many different families of chaperones; each family acts to aid protein folding in a different way. In bacteria like E. coli, many of these proteins are highly expressed under conditions of high stress, for example, when the bacterium is placed in high temperatures, thus heat shock protein chaperones are the most extensive.
A variety of nomenclatures are in use for chaperones. As heat shock proteins, the names are classically formed by "Hsp" followed by the approximate molecular mass in kilodaltons; such names are commonly used for eukaryotes such as yeast. The bacterial names have more varied forms, and refer directly to their apparent function at discovery. For example, "GroEL" originally stands for "phage growth defect, overcome by mutation in phage gene E, large subunit".[25]
Hsp10 and Hsp60
Hsp10/60 (GroEL/GroES complex in E. coli) is the best characterized large (~ 1 MDa) chaperone complex. GroEL (Hsp60) is a double-ring 14mer with a hydrophobic patch at its opening; it is so large it can accommodate native folding of 54-kDa GFP in its lumen. GroES (Hsp10) is a single-ring heptamer that binds to GroEL in the presence of ATP or ADP. GroEL/GroES may not be able to undo previous aggregation, but it does compete in the pathway of misfolding and aggregation.[26] Also acts in mitochondrial matrix as molecular chaperone.
Hsp70 and Hsp40
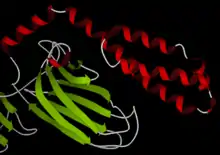
Hsp70 (DnaK in E. coli) is perhaps the best characterized small (~ 70 kDa) chaperone. The Hsp70 proteins are aided by Hsp40 proteins (DnaJ in E. coli), which increase the ATP consumption rate and activity of the Hsp70s. The two protein are named "Dna" in bacteria because they were initially identified as being required for E. coli DNA replication.[27]
It has been noted that increased expression of Hsp70 proteins in the cell results in a decreased tendency toward apoptosis. Although a precise mechanistic understanding has yet to be determined, it is known that Hsp70s have a high-affinity bound state to unfolded proteins when bound to ADP, and a low-affinity state when bound to ATP.
It is thought that many Hsp70s crowd around an unfolded substrate, stabilizing it and preventing aggregation until the unfolded molecule folds properly, at which time the Hsp70s lose affinity for the molecule and diffuse away.[28] Hsp70 also acts as a mitochondrial and chloroplastic molecular chaperone in eukaryotes.
Hsp90
Hsp90 (HtpG in E. coli[lower-alpha 1]) may be the least understood chaperone. Its molecular weight is about 90 kDa, and it is necessary for viability in eukaryotes (possibly for prokaryotes as well). Heat shock protein 90 (Hsp90) is a molecular chaperone essential for activating many signaling proteins in the eukaryotic cell.
Each Hsp90 has an ATP-binding domain, a middle domain, and a dimerization domain. Originally thought to clamp onto their substrate protein (also known as a client protein) upon binding ATP, the recently published structures by Vaughan et al. and Ali et al. indicate that client proteins may bind externally to both the N-terminal and middle domains of Hsp90.[29][30]
Hsp90 may also require co-chaperones-like immunophilins, Sti1, p50 (Cdc37), and Aha1, and also cooperates with the Hsp70 chaperone system.[31][32]
Hsp100
Hsp100 (Clp family in E. coli) proteins have been studied in vivo and in vitro for their ability to target and unfold tagged and misfolded proteins.
Proteins in the Hsp100/Clp family form large hexameric structures with unfoldase activity in the presence of ATP. These proteins are thought to function as chaperones by processively threading client proteins through a small 20 Å (2 nm) pore, thereby giving each client protein a second chance to fold.
Some of these Hsp100 chaperones, like ClpA and ClpX, associate with the double-ringed tetradecameric serine protease ClpP; instead of catalyzing the refolding of client proteins, these complexes are responsible for the targeted destruction of tagged and misfolded proteins.
Hsp104, the Hsp100 of Saccharomyces cerevisiae, is essential for the propagation of many yeast prions. Deletion of the HSP104 gene results in cells that are unable to propagate certain prions.
Bacteriophage
The genes of bacteriophage (phage) T4 that encode proteins with a role in determining phage T4 structure were identified using conditional lethal mutants.[33] Most of these proteins proved to be either major or minor structural components of the completed phage particle. However among the gene products (gps) necessary for phage assembly, Snustad[34] identified a group of gps that act catalytically rather than being incorporated themselves into the phage structure. These gps were gp26, gp31, gp38, gp51, gp28, and gp4 [gene 4 is synonymous with genes 50 and 65, and thus the gp can be designated gp4(50)(65)]. The first four of these six gene products have since been recognized as being chaperone proteins. Additionally, gp40, gp57A, gp63 and gpwac have also now been identified as chaperones.
Phage T4 morphogenesis is divided into three independent pathways: the head, the tail and the long tail fiber pathways as detailed by Yap and Rossman.[35] With regard to head morphogenesis, chaperone gp31 interacts with the bacterial host chaperone GroEL to promote proper folding of the major head capsid protein gp23.[36][35] Chaperone gp40 participates in the assembly of gp20, thus aiding in the formation of the connector complex that initiates head procapsid assembly.[36][35] Gp4(50)(65), although not specifically listed as a chaperone, acts catalytically as a nuclease that appears to be essential for morphogenesis by cleaving packaged DNA to enable the joining of heads to tails.[37]
During overall tail assembly, chaperone proteins gp26 and gp51 are necessary for baseplate hub assembly.[38] Gp57A is required for correct folding of gp12, a structural component of the baseplate short tail fibers.[38]
Synthesis of the long tail fibers depends on the chaperone protein gp57A that is needed for the trimerization of gp34 and gp37, the major structural proteins of the tail fibers.[36][35] The chaperone protein gp38 is also required for the proper folding of gp37.[38][39] Chaperone proteins gp63 and gpwac are employed in attachment of the long tail fibers to the tail baseplate.[38]
History
The investigation of chaperones has a long history.[40] The term "molecular chaperone" appeared first in the literature in 1978, and was invented by Ron Laskey to describe the ability of a nuclear protein called nucleoplasmin to prevent the aggregation of folded histone proteins with DNA during the assembly of nucleosomes.[41] The term was later extended by R. John Ellis in 1987 to describe proteins that mediated the post-translational assembly of protein complexes.[42] In 1988, it was realised that similar proteins mediated this process in both prokaryotes and eukaryotes.[43] The details of this process were determined in 1989, when the ATP-dependent protein folding was demonstrated in vitro.[44]
Clinical significance
There are many disorders associated with mutations in genes encoding chaperones (i.e. multisystem proteinopathy) that can affect muscle, bone and/or the central nervous system.[45]
Notes
- Initially identified as Drosophilia Hsp83 homologue. Name stands for "high temperature protein G".
References
- Richardson RT, Alekseev OM, Grossman G, Widgren EE, Thresher R, Wagner EJ, et al. (July 2006). "Nuclear autoantigenic sperm protein (NASP), a linker histone chaperone that is required for cell proliferation". The Journal of Biological Chemistry. 281 (30): 21526–34. doi:10.1074/jbc.M603816200. PMID 16728391.
- Alekseev OM, Richardson RT, Alekseev O, O'Rand MG (May 2009). "Analysis of gene expression profiles in HeLa cells in response to overexpression or siRNA-mediated depletion of NASP". Reproductive Biology and Endocrinology. 7: 45. doi:10.1186/1477-7827-7-45. PMC 2686705. PMID 19439102.
- [Chaperone Action at the Single-Molecule Level http://pubs.acs.org/doi/abs/10.1021/cr400326k]
- Ellis RJ, van der Vies SM (1991). "Molecular chaperones". Annual Review of Biochemistry. 60: 321–347. doi:10.1146/annurev.bi.60.070191.001541. PMID 1679318.
- Bascos NA, Landry SJ (December 2019). "A History of Molecular Chaperone Structures in the Protein Data Bank". International Journal of Molecular Sciences. 20 (24): 6195. doi:10.3390/ijms20246195. PMC 6940948. PMID 31817979.
- Hoffmann JH, Linke K, Graf PC, Lilie H, Jakob U (January 2004). "Identification of a redox-regulated chaperone network". The EMBO Journal. 23 (1): 160–8. doi:10.1038/sj.emboj.7600016. PMC 1271656. PMID 14685279.
- Nillegoda NB, Kirstein J, Szlachcic A, Berynskyy M, Stank A, Stengel F, et al. (August 2015). "Crucial HSP70 co-chaperone complex unlocks metazoan protein disaggregation". Nature. 524 (7564): 247–51. Bibcode:2015Natur.524..247N. doi:10.1038/nature14884. PMC 4830470. PMID 26245380.
- Balchin D, Hayer-Hartl M, Hartl FU (July 2016). "In vivo aspects of protein folding and quality control". Science. 353 (6294): aac4354. doi:10.1126/science.aac4354. hdl:11858/00-001M-0000-002B-0856-C. PMID 27365453. S2CID 5174431.
- van den Berg B, Wain R, Dobson CM, Ellis RJ (August 2000). "Macromolecular crowding perturbs protein refolding kinetics: implications for folding inside the cell". The EMBO Journal. 19 (15): 3870–5. doi:10.1093/emboj/19.15.3870. PMC 306593. PMID 10921869.
- van den Berg B, Ellis RJ, Dobson CM (December 1999). "Effects of macromolecular crowding on protein folding and aggregation". The EMBO Journal. 18 (24): 6927–33. doi:10.1093/emboj/18.24.6927. PMC 1171756. PMID 10601015.
- Ellis RJ, Minton AP (May 2006). "Protein aggregation in crowded environments". Biological Chemistry. 387 (5): 485–97. doi:10.1515/BC.2006.064. PMID 16740119. S2CID 7336464.
- Martin J, Hartl FU (February 1997). "The effect of macromolecular crowding on chaperonin-mediated protein folding". Proceedings of the National Academy of Sciences of the United States of America. 94 (4): 1107–12. Bibcode:1997PNAS...94.1107M. doi:10.1073/pnas.94.4.1107. PMC 19752. PMID 9037014.
- Ellis RJ (2007). "Protein Misassembly". Molecular Aspects of the Stress Response: Chaperones, Membranes and Networks. Advances in Experimental Medicine and Biology. Vol. 594. New York, N.Y.: Sprinter Science+Business Media, LLC ; Austin, Tex.: Landes Bioscience/Eurekah.com. pp. 1–13. doi:10.1007/978-0-387-39975-1_1. ISBN 978-0-387-39974-4. PMID 17205670.
- Pauwels K, Van Molle I, Tommassen J, Van Gelder P (May 2007). "Chaperoning Anfinsen: the steric foldases" (PDF). Molecular Microbiology. 64 (4): 917–22. doi:10.1111/j.1365-2958.2007.05718.x. PMID 17501917. S2CID 6435829. Archived from the original (PDF) on 2012-05-23.
- Zhou J, Xu Z (October 2005). "The structural view of bacterial translocation-specific chaperone SecB: implications for function" (PDF). Molecular Microbiology. 58 (2): 349–57. doi:10.1111/j.1365-2958.2005.04842.x. hdl:2027.42/74325. PMID 16194224. S2CID 33227532.
- Specht S, Miller SB, Mogk A, Bukau B (14 November 2011). "Hsp42 is required for sequestration of protein aggregates into deposition sites in Saccharomyces cerevisiae". J. Cell Biol. 195 (4): 617–29. doi:10.1083/jcb.201106037. PMC 3257523. PMID 22065637.
- Ojha J, Masilamoni G, Dunlap D, Udoff RA, Cashikar AG (August 2011). "Sequestration of toxic oligomers by HspB1 as a cytoprotective mechanism". Mol. Cell. Biol. 31 (15): 3146–57. doi:10.1128/MCB.01187-10. PMC 3147607. PMID 21670152.
- Mannini B, Cascella R, Zampagni M, van Waarde-Verhagen M, Meehan S, Roodveldt C, Campioni S, Boninsegna M, Penco A, Relini A, Kampinga HH, Dobson CM, Wilson MR, Cecchi C, Chiti F (31 July 2012). "Molecular mechanisms used by chaperones to reduce the toxicity of aberrant protein oligomers". Proc. Natl. Acad. Sci. USA. 109 (31): 12479–84. Bibcode:2012PNAS..10912479M. doi:10.1073/pnas.1117799109. PMC 3411936. PMID 22802614.
- Sadigh-Eteghad S, Majdi A, Talebi M, Mahmoudi J, Babri S (May 2015). "Regulation of nicotinic acetylcholine receptors in Alzheimer׳s disease: a possible role of chaperones". European Journal of Pharmacology. 755: 34–41. doi:10.1016/j.ejphar.2015.02.047. PMID 25771456. S2CID 31929001.
- Salamanca HH, Antonyak MA, Cerione RA, Shi H, Lis JT (2014). "Inhibiting heat shock factor 1 in human cancer cells with a potent RNA aptamer". PLOS ONE. 9 (5): e96330. Bibcode:2014PLoSO...996330S. doi:10.1371/journal.pone.0096330. PMC 4011729. PMID 24800749.
- Finka A, Goloubinoff P (September 2013). "Proteomic data from human cell cultures refine mechanisms of chaperone-mediated protein homeostasis". Cell Stress & Chaperones. 18 (5): 591–605. doi:10.1007/s12192-013-0413-3. PMC 3745260. PMID 23430704.
- Ruoppolo M, Orrù S, Talamo F, Ljung J, Pirneskoski A, Kivirikko KI, et al. (May 2003). "Mutations in domain a' of protein disulfide isomerase affect the folding pathway of bovine pancreatic ribonuclease A". Protein Science. 12 (5): 939–52. doi:10.1110/ps.0242803. PMC 2323865. PMID 12717017.
- Soluble complexes of target proteins and peptidyl prolyl isomerase ...
- Frickel EM, Riek R, Jelesarov I, Helenius A, Wuthrich K, Ellgaard L (February 2002). "TROSY-NMR reveals interaction between ERp57 and the tip of the calreticulin P-domain". Proceedings of the National Academy of Sciences of the United States of America. 99 (4): 1954–9. Bibcode:2002PNAS...99.1954F. doi:10.1073/pnas.042699099. PMC 122301. PMID 11842220.
- Smith, Tracy (1 December 1999). "The discovery of chaperonins". Nature Structural Biology. 6 (12): 1090. doi:10.1038/70015. PMID 10581544. S2CID 6158370.
- Fenton WA, Horwich AL (May 2003). "Chaperonin-mediated protein folding: fate of substrate polypeptide". Quarterly Reviews of Biophysics. 36 (2): 229–56. doi:10.1017/S0033583503003883. PMID 14686103. S2CID 10328521.
- Yochem, J; Uchida, H; Sunshine, M; Saito, H; Georgopoulos, CP; Feiss, M (4 August 1978). "Genetic analysis of two genes, dnaJ and dnaK, necessary for Escherichia coli and bacteriophage lambda DNA replication". Molecular & General Genetics. 164 (1): 9–14. doi:10.1007/BF00267593. PMID 360041. S2CID 28144214.
- Mayer MP, Bukau B (March 2005). "Hsp70 chaperones: cellular functions and molecular mechanism". Cellular and Molecular Life Sciences. 62 (6): 670–84. doi:10.1007/s00018-004-4464-6. PMC 2773841. PMID 15770419.
- Vaughan CK, Gohlke U, Sobott F, Good VM, Ali MM, Prodromou C, et al. (September 2006). "Structure of an Hsp90-Cdc37-Cdk4 complex". Molecular Cell. 23 (5): 697–707. doi:10.1016/j.molcel.2006.07.016. PMC 5704897. PMID 16949366.
- Ali MM, Roe SM, Vaughan CK, Meyer P, Panaretou B, Piper PW, et al. (April 2006). "Crystal structure of an Hsp90-nucleotide-p23/Sba1 closed chaperone complex". Nature. 440 (7087): 1013–7. Bibcode:2006Natur.440.1013A. doi:10.1038/nature04716. PMC 5703407. PMID 16625188.
- Terasawa K, Minami M, Minami Y (April 2005). "Constantly updated knowledge of Hsp90". Journal of Biochemistry. 137 (4): 443–7. doi:10.1093/jb/mvi056. PMID 15858167.
- Pearl LH, Prodromou C (2006). "Structure and mechanism of the Hsp90 molecular chaperone machinery". Annual Review of Biochemistry. 75: 271–94. doi:10.1146/annurev.biochem.75.103004.142738. PMID 16756493.
- Edgar RS, Epstein RH. The genetics of a bacterial virus. Sci Am. 1965;212:70-78. doi:10.1038/scientificamerican0265-70
- Snustad DP. Dominance interactions in Escherichia coli cells mixedly infected with bacteriophage T4D wild-type and amber mutants and their possible implications as to type of gene-product function: catalytic vs. stoichiometric. Virology. 1968;35(4):550-563. doi:10.1016/0042-6822(68)90285-7
- Yap ML, Rossmann MG. Structure and function of bacteriophage T4. Future Microbiol. 2014;9(12):1319-1327. doi:10.2217/fmb.14.91
- Marusich EI, Kurochkina LP, Mesyanzhinov VV. Chaperones in bacteriophage T4 assembly. Biochemistry (Mosc). 1998;63(4):399-406
- Benler S, Hung SH, Vander Griend JA, Peters GA, Rohwer F, Segall AM. Gp4 is a nuclease required for morphogenesis of T4-like bacteriophages. Virology. 2020;543:7-12. doi:10.1016/j.virol.2020.01.008
- Leiman PG, Arisaka F, van Raaij MJ, et al. Morphogenesis of the T4 tail and tail fibers. Virol J. 2010;7:355. Published 2010 Dec 3. doi:10.1186/1743-422X-7-355
- Hyman P, van Raaij M. Bacteriophage T4 long tail fiber domains. Biophys Rev. 2018;10(2):463-471. doi:10.1007/s12551-017-0348-5
- Ellis RJ (September 1996). "Discovery of molecular chaperones". Cell Stress & Chaperones. 1 (3): 155–60. PMC 248474. PMID 9222600.
- Laskey RA, Honda BM, Mills AD, Finch JT (October 1978). "Nucleosomes are assembled by an acidic protein which binds histones and transfers them to DNA". Nature. 275 (5679): 416–20. Bibcode:1978Natur.275..416L. doi:10.1038/275416a0. PMID 692721. S2CID 2535641.
- Ellis J (1987). "Proteins as molecular chaperones". Nature. 328 (6129): 378–9. Bibcode:1987Natur.328..378E. doi:10.1038/328378a0. PMID 3112578. S2CID 4337273.
- Hemmingsen SM, Woolford C, van der Vies SM, Tilly K, Dennis DT, Georgopoulos CP, et al. (May 1988). "Homologous plant and bacterial proteins chaperone oligomeric protein assembly". Nature. 333 (6171): 330–4. Bibcode:1988Natur.333..330H. doi:10.1038/333330a0. PMID 2897629. S2CID 4325057.
- Goloubinoff P, Christeller JT, Gatenby AA, Lorimer GH (1989). "Reconstitution of active dimeric ribulose bisphosphate carboxylase from an unfoleded state depends on two chaperonin proteins and Mg-ATP". Nature. 342 (6252): 884–9. Bibcode:1989Natur.342..884G. doi:10.1038/342884a0. PMID 10532860. S2CID 4319510.
- Taylor JP (August 2015). "Multisystem proteinopathy: intersecting genetics in muscle, bone, and brain degeneration". Neurology. 85 (8): 658–60. doi:10.1212/WNL.0000000000001862. PMID 26208960. S2CID 42203997.